EUKARYOTES AND THE ORIGIN OF THE EUKARYOTIC CELL
Eukaryotic cells, in general, are bigger and more elaborate than bacterial and archaeal cells, and their genomes are bigger and more elaborate, too. The greater cell size is accompanied by radical differences in cell structure and function: in particular, eukaryotes contain a diverse set of intracellular organelles—discrete membrane-enclosed subcompartments and large membraneless macromolecular assemblies—each with a distinct composition and function. Some eukaryotic cells live independent lives as single-cell organisms. Others live in multicellular assemblies—indeed, all of the more complex multicellular organisms on Earth, including plants, animals, and fungi, are formed from eukaryotic cells.
We begin by discussing how eukaryotic cells are organized and how they might have evolved from more ancient prokaryotes. We then briefly consider how eukaryotic genomes differ from those of prokaryotes, as well as how the cells in multicellular organisms become differently specialized as an embryo develops, so as to contribute to the welfare of the organism as a whole.
Eukaryotic Cells Contain a Variety of Organelles
By definition, eukaryotic cells keep almost all their DNA in a membrane-enclosed internal compartment—the nucleus, which is usually the most conspicuous organelle (Figure 1–21). The long DNA polymers in the nucleus are packaged with proteins to form chromosomes, which only become visible in a light microscope when they condense in preparation for cell division. The nuclear envelope, a double layer of membrane, surrounds the nucleus and separates the nuclear DNA from the cytoplasm, which, in a eukaryotic cell, includes everything between the plasma membrane and the nucleus. As shown in the figure, the nuclear envelope is perforated by nuclear pores, which are channels formed by protein complexes that mediate the two-way traffic of large molecules between the nucleus and the cytoplasm.
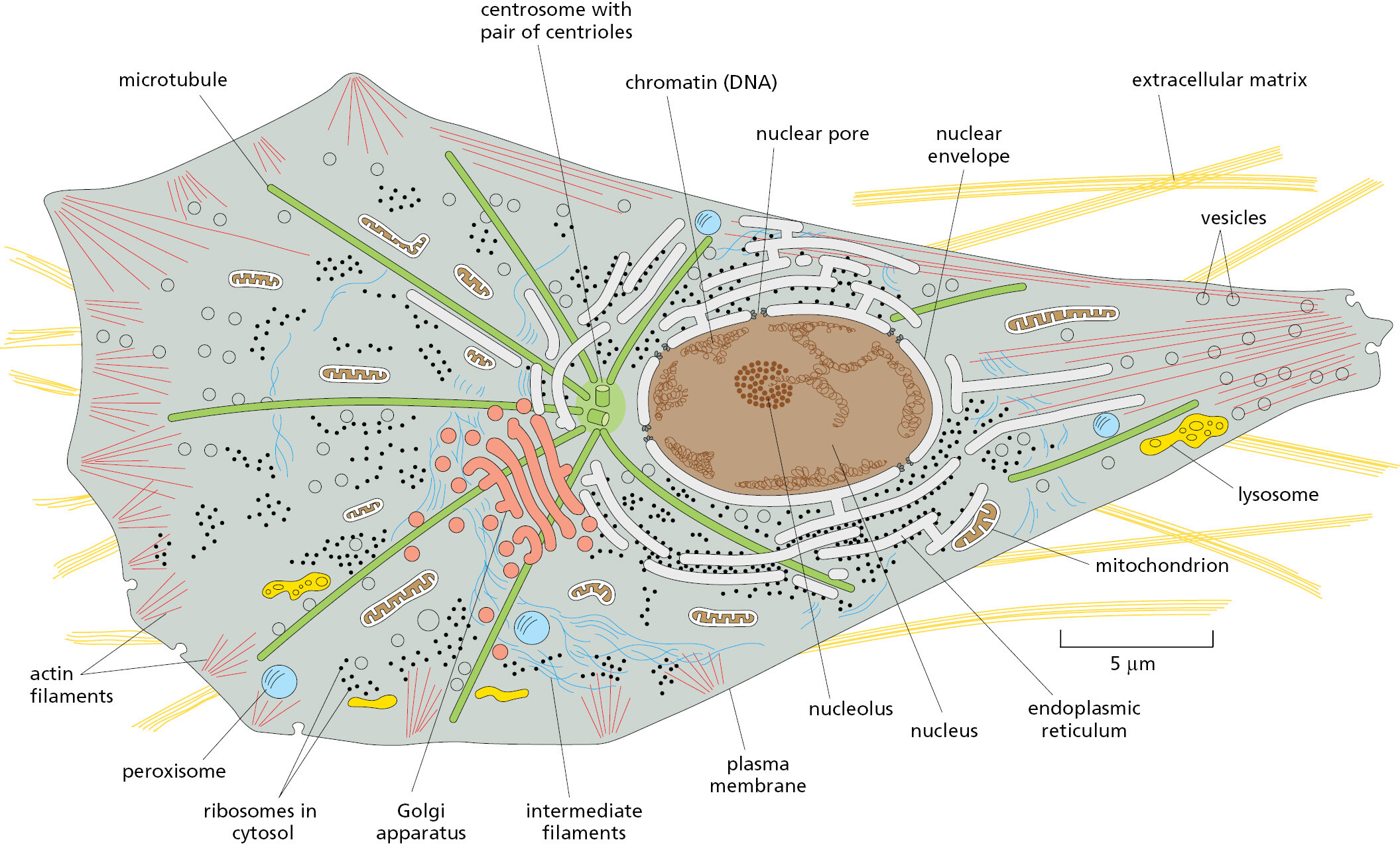
Eukaryotic cells have many other features that set them apart from bacterial and archaeal cells. They are typically 10–30 times bigger in linear dimension and 1000–10,000 times larger in volume than a typical prokaryotic cell. They have an elaborate cytoskeleton in the cytoplasm, consisting of several types of protein filaments (see Figure 1–21) that, together with the many proteins that attach to them, form a network of girders, ropes, and motors that gives the cell mechanical strength and performs various other functions: when the cell divides, for example, the cytoskeleton reorganizes and pulls the replicated chromosomes apart and distributes them equally to the two daughter cells. In the case of animal cells and some free-living, single-cell eukaryotes, the cytoskeleton controls cell shape and drives and guides cell movements (Movie 1.1). Lacking the kind of tough cell wall characteristic of bacteria and archaea, these eukaryotic cells can change their shape rapidly, in some cases enabling them to move and engulf other cells and small objects by a process called phagocytosis (Figure 1–22).
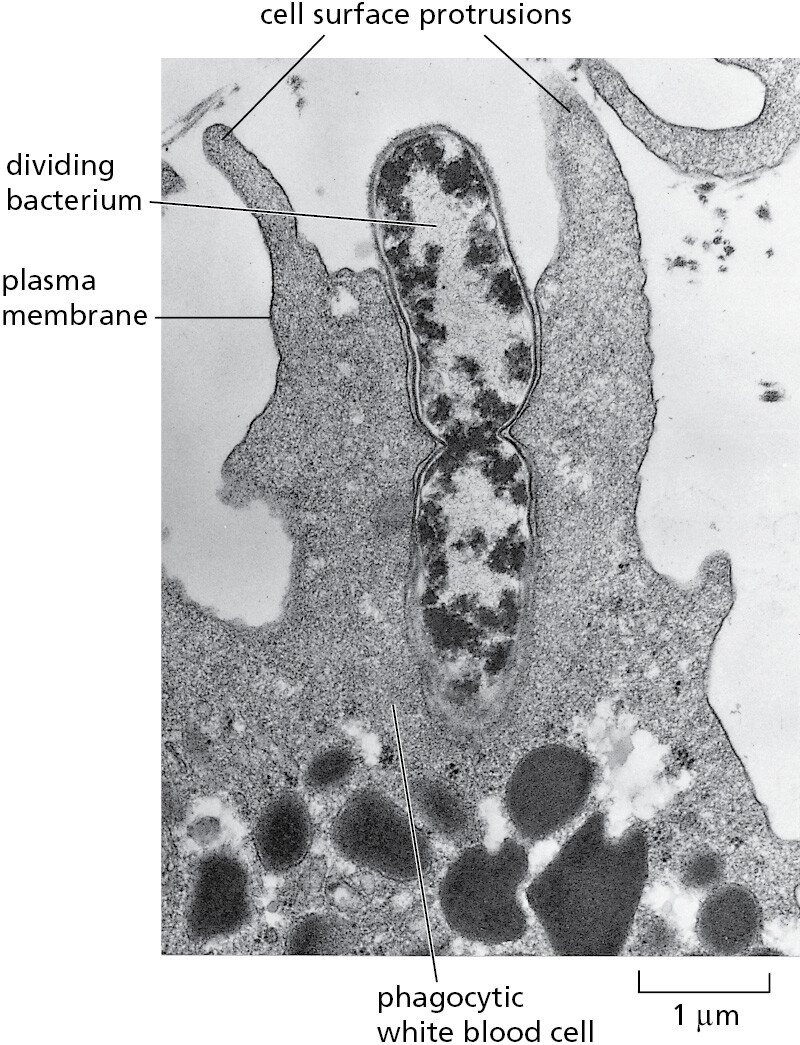
There are many other membrane-enclosed organelles in eukaryotic cells. Unlike the nucleus, most of them are enclosed by single membranes. The most extensive organelle is the endoplasmic reticulum (ER), which is where most cell membrane components are made, along with materials destined for secretion to the outside of the cell. The Golgi apparatus receives these molecules from the ER and modifies and packages them for secretion or transport to another cell compartment. Lysosomes are small irregularly shaped organelles in which intracellular digestion occurs. Peroxisomes are small vesicles where hydrogen peroxide is used to inactivate toxic molecules.
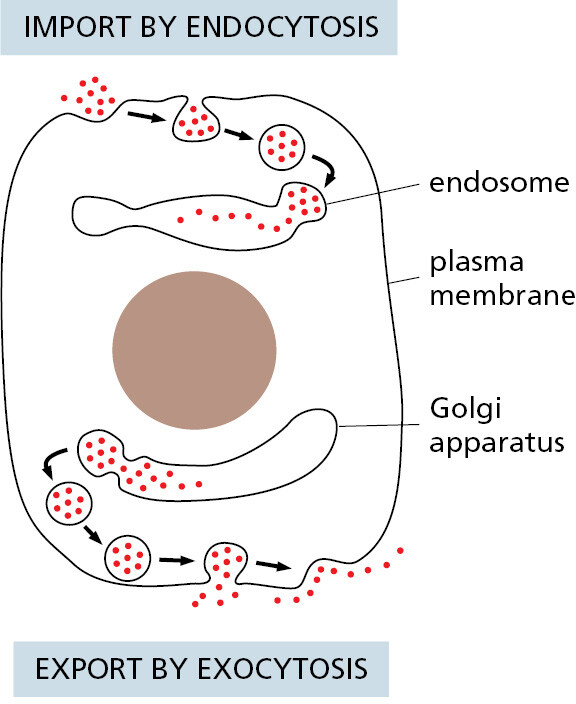
A continual exchange of materials occurs between these single-membrane-enclosed organelles, mediated mainly by small transport vesicles that pinch off from the membrane of one organelle and fuse with that of another. To connect the eukaryotic cell with its surroundings, a similar vesicle-mediated exchange goes on continually at the cell surface. Here, portions of the plasma membrane pinch in to form intracellular vesicles that carry material captured from the external medium into the cell—a process called endocytosis; and in the reverse process, called exocytosis, vesicles from inside the cell fuse with the plasma membrane and release their contents to the exterior (Figure 1–23).
Besides the nucleus, there are two other eukaryotic cell organelles that are enclosed in double membranes—mitochondria and, in plant cells and algae, chloroplasts. Mitochondria take up oxygen and harness energy from the oxidation of food molecules, such as sugars and fats, to produce most of the ATP (adenosine triphosphate) that powers the cell’s activities. Chloroplasts perform photosynthesis in plant cells and algae, using the energy of sunlight to synthesize carbohydrates from atmospheric CO2 and water, delivering these energy-rich products to the host cell as food. In many eukaryotic cells, roughly half of the cytoplasm is occupied by membrane-enclosed organelles. The surrounding fluid is called the cytosol. It contains ribosomes, which translate RNAs into proteins, and it is also where most of the cell’s other metabolic reactions take place.
In addition to the membrane-enclosed organelles just described, eukaryotic cells contain a variety of smaller organelles that lack membranes. Instead, multiple components that work together are held in close proximity in biomolecular condensates, which will be described in Chapters 3, 6, and 12. An example is the nucleolus, where ribosome assembly takes place (see Figure 1–21). Figure 1–24 summarizes the membrane-enclosed organelles in eukaryotic cells, each of which will be discussed in detail in later chapters.
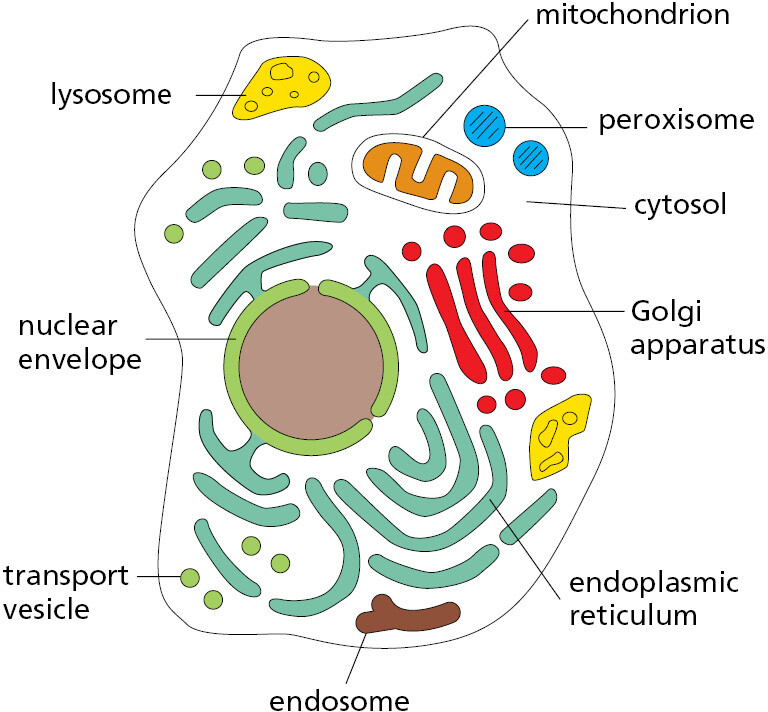
Mitochondria Evolved from a Symbiotic Bacterium Captured by an Ancient Archaeon
A fundamental question in both evolution and cell biology is how did the first eukaryotic cell arise? The evidence suggests that it happened when an archaeal and a bacterial cell merged about 2 billion years ago, in a world that had contained only prokaryotes for more than 1.5 billion years.
All eukaryotic cells contain (or at one time did contain) mitochondria (Figure 1–25). Mitochondria are similar in size to small bacteria, and both reproduce by dividing. The mitochondria contain their own DNA, with genes that resemble bacterial genes; they also contain their own ribosomes and translation factors that resemble those in bacteria. These and other similarities between mitochondria and present-day bacteria provide strong evidence that mitochondria evolved from an aerobic bacterium (one that harvested energy by combining electrons derived from foodstuffs with oxygen gas) that was captured by an anaerobic cell. These two cells (and their descendants) were then able to evolve an endosymbiotic relationship, providing mutual metabolic support within a common cytoplasm.
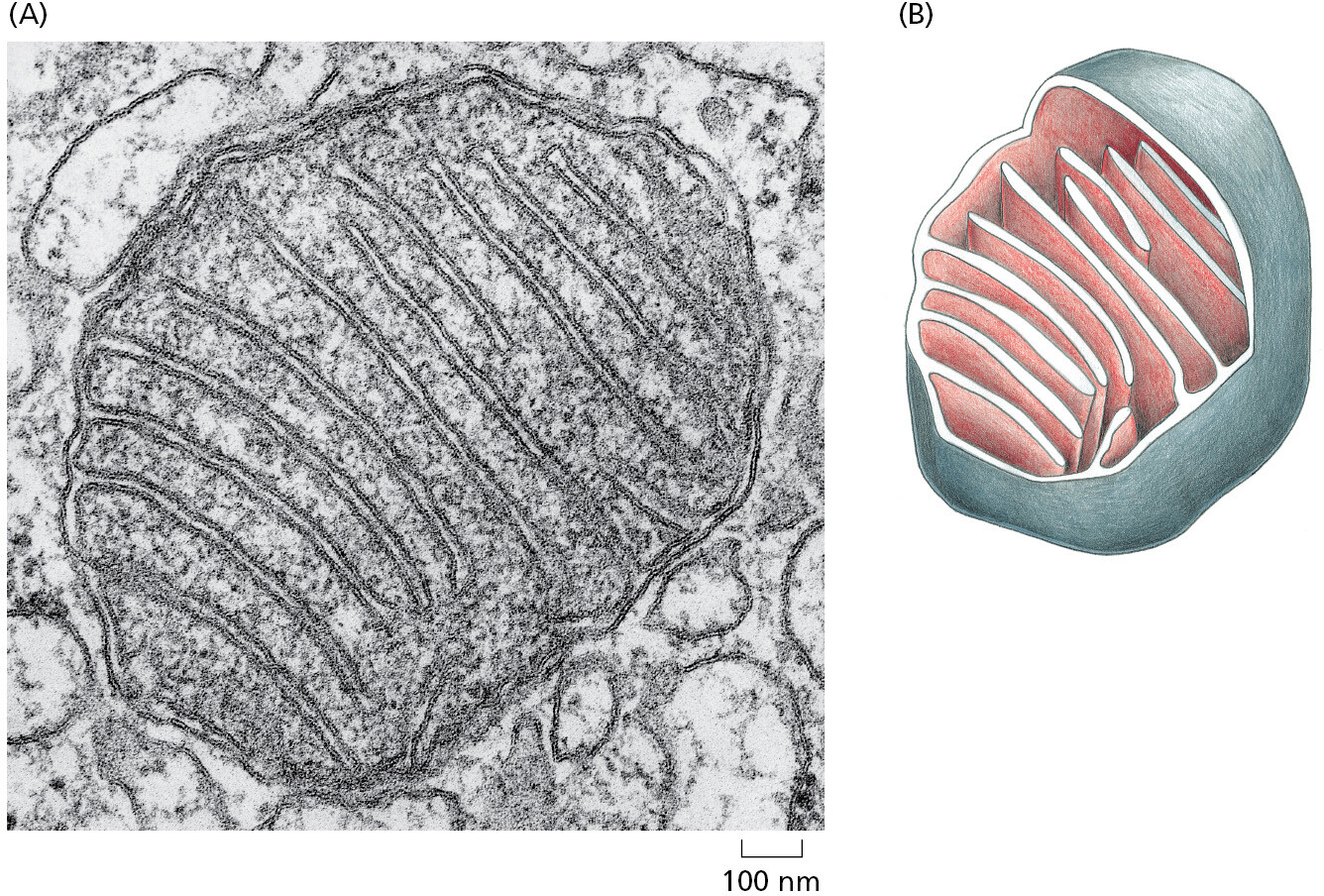
There are also good reasons to believe that the ancestral capturing cell was an archaeon. As we have seen, the genomes of present-day archaea encode many proteins that are characteristic of present-day eukaryotic cells. Those archaea with the most eukaryotic-like genes belong to the Asgard lineage, first identified by sequencing DNA fragments obtained from the seabed. But no living example had been seen or cultivated—until very recently, when, after a heroic 12-year-long isolation procedure, the first Asgard archaeon was propagated in culture. This remarkable, living, anaerobic archaeon looked like no other prokaryote—having long branching protrusions—and it seemed to live in an ectosymbiotic relationship with another bacterium and another archaeon, both of which were isolated with it (Figure 1–26). The discovery of the strange Asgard archaeon provides a glimpse of how an ancient archaeon might eventually have captured an aerobic bacterium to initiate the eukaryotic lineage, with a hypothetical pathway being illustrated in Figure 1–27 and discussed in detail in Chapter 12.
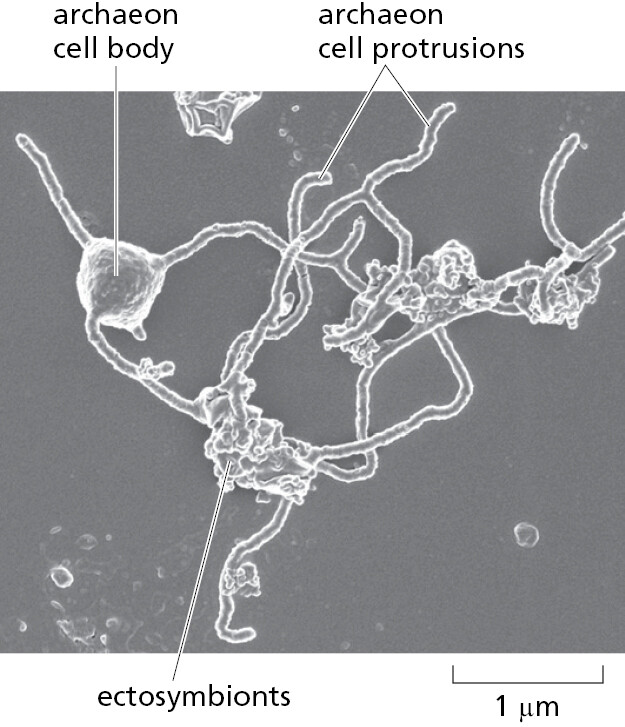
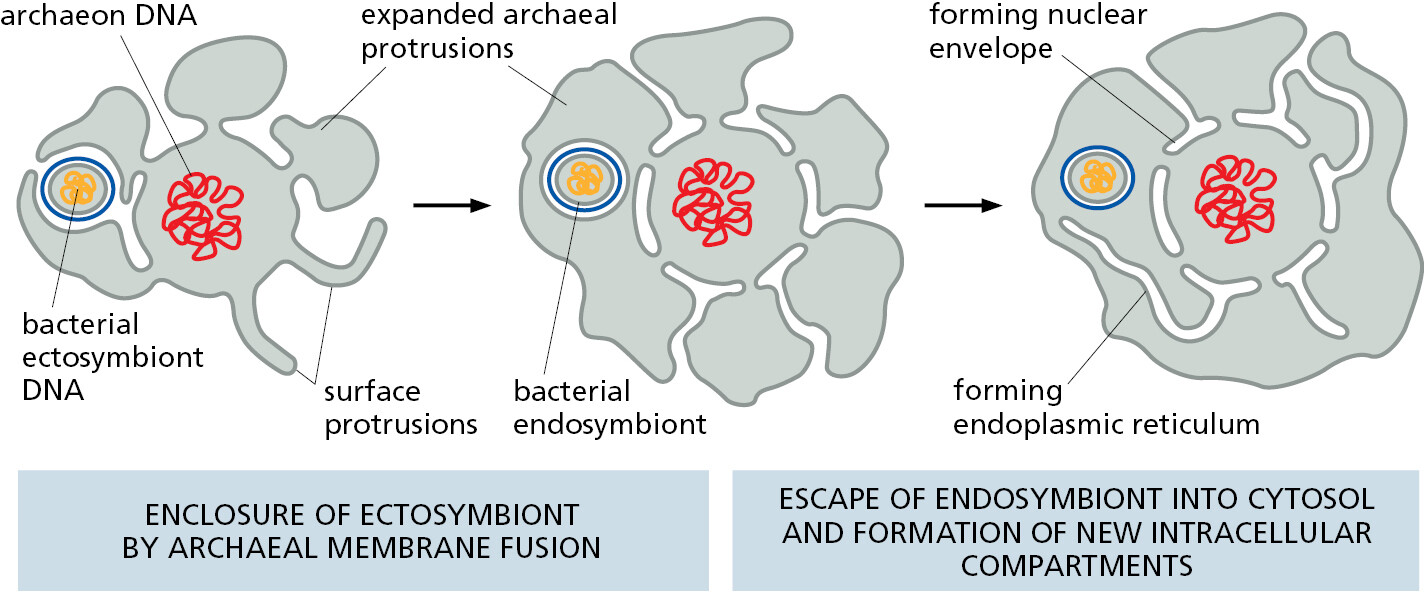
Chloroplasts Evolved from a Symbiotic Photosynthetic Bacterium Engulfed by an Ancient Eukaryotic Cell
Chloroplasts (Figure 1–28) perform photosynthesis in plant cells and algae, using the energy of sunlight to synthesize their own “food” (in the form of carbohydrates) from atmospheric CO2 and water. Like mitochondria, they are enclosed in double membranes, have their own “circular” genomes, and reproduce by dividing. They almost certainly evolved from a symbiotic photosynthetic bacterium that was captured by an ancient eukaryotic cell that already possessed mitochondria. This bacterium may have been captured by phagocytosis, a frequent process in eukaryotes (see Figure 1–22).
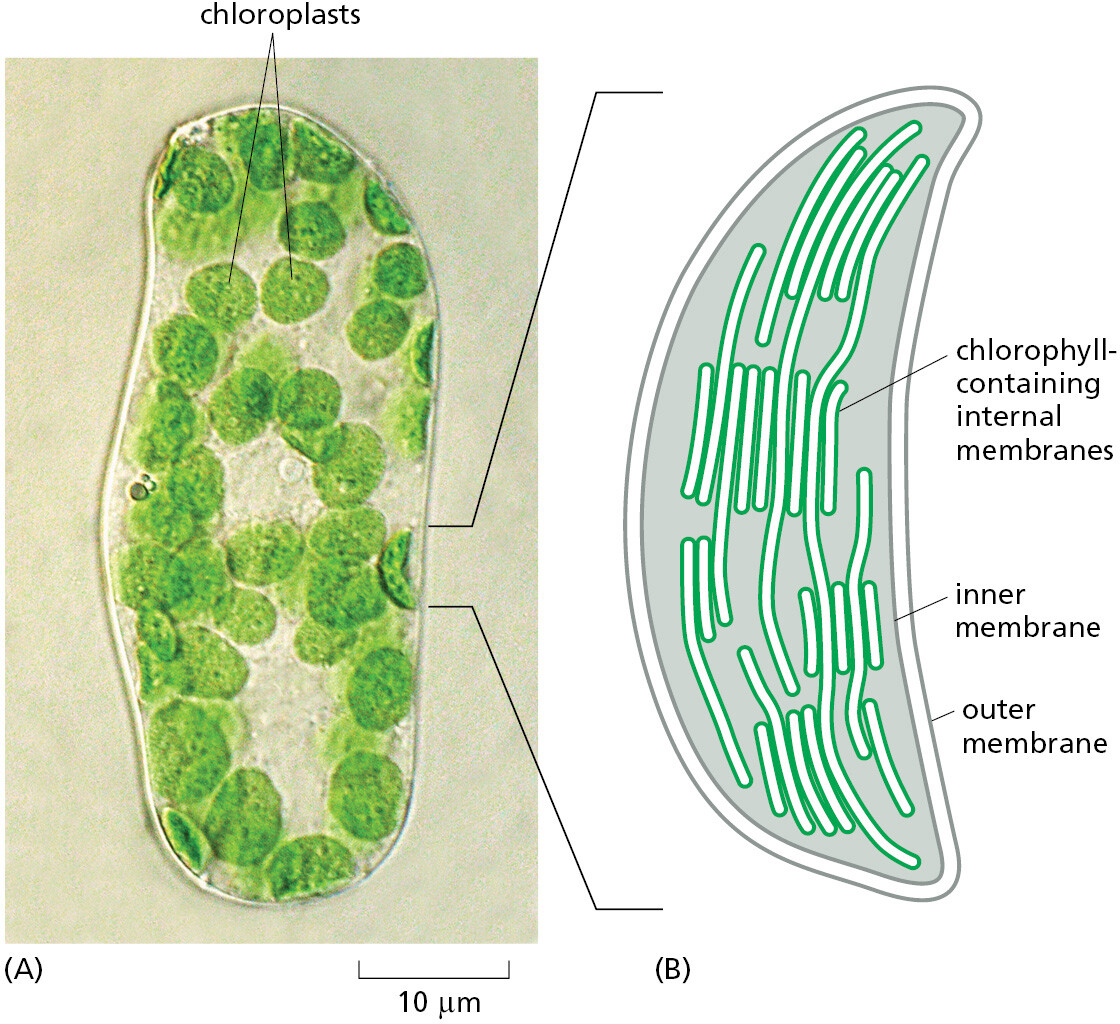
Whereas some single-cell eukaryotes are hunters that live by capturing other cells and eating them (see Figure 1–34), a eukaryotic cell equipped with chloroplasts has no need to chase after other cells as prey; it is nourished by the captive chloroplasts it has inherited from its ancient eukaryotic ancestors. Correspondingly, plant cells, although they possess the cytoskeletal equipment for movement, have lost the ability to change shape rapidly and to engulf other cells by phagocytosis. Instead, they wrap themselves in a tough, protective cell wall. If some eukaryotic cells can be viewed as hunters, then one might view plant cells as having given up hunting for farming.
Fungi represent yet another eukaryotic way of life. Fungal cells, like animal cells, possess mitochondria but not chloroplasts; they have a tough outer wall that limits their ability to move rapidly or to take up other cells. Fungi, it seems, have turned from hunters into scavengers. Other cells secrete nutrient molecules or release them after death, and fungi feed on these leavings—often performing whatever digestion is necessary extracellularly, by secreting digestive enzymes to the exterior.
Eukaryotes Have Hybrid Genomes
As just discussed, the genetic information of eukaryotic cells has a hybrid origin—from an ancestral anaerobic archaeon and from the bacteria it adopted as endosymbionts (Figure 1–29). Most of this genetic information (DNA) is stored in the nucleus, although a small amount remains inside the organelles that evolved from the captured bacteria—the mitochondria and, in plant and algal cells, the chloroplasts. When the mitochondrial DNA and the chloroplast DNA are separated from the nuclear DNA and individually sequenced, both the mitochondrial and chloroplast genomes are found to be cut-down versions of the corresponding bacterial genomes: in a human cell, for example, the mitochondrial genome consists of only 16,569 nucleotide pairs, and it codes for only 13 proteins plus a set of 24 RNAs involved in protein synthesis.
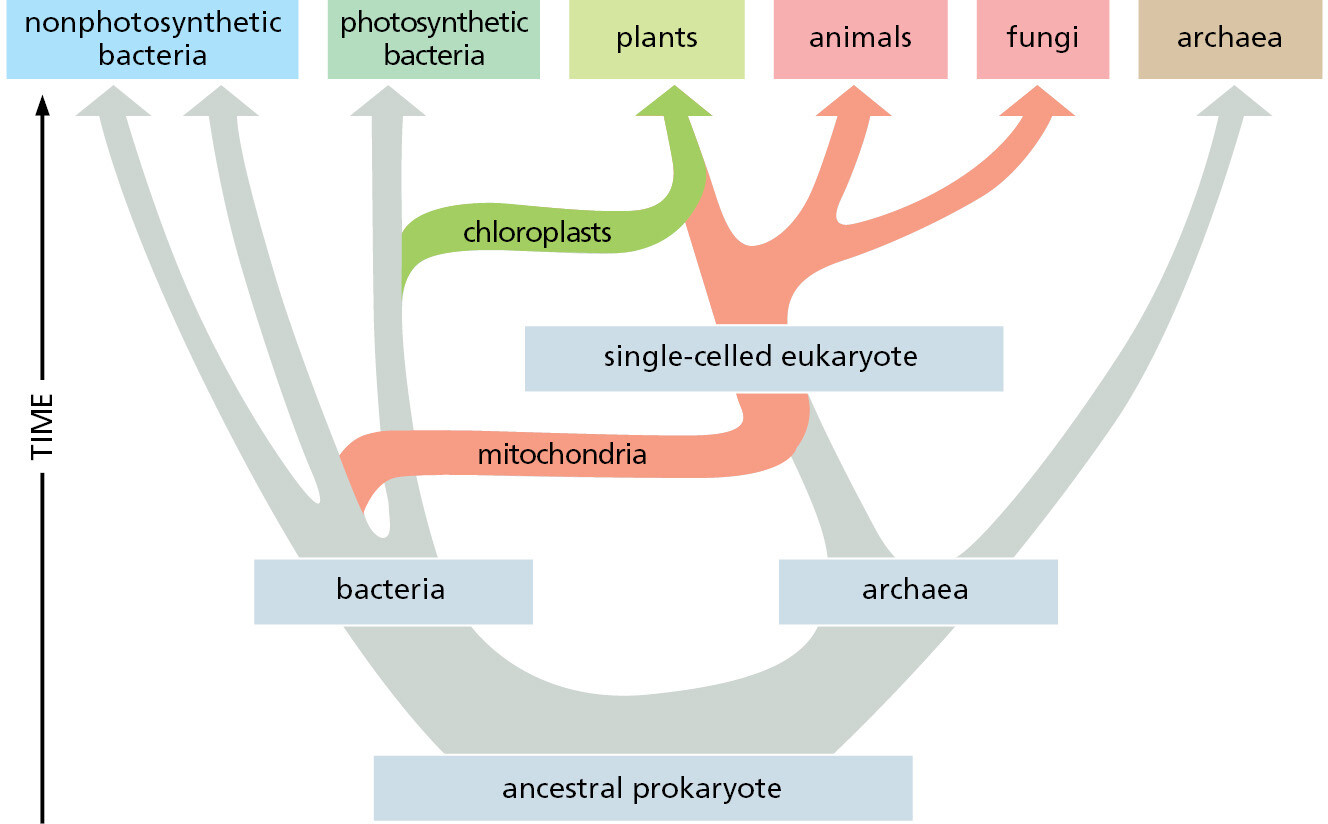
Many of the genes that are missing from the mitochondria and chloroplasts have not been lost; instead, they have moved from the endosymbiont genomes into the DNA of the host-cell nucleus. Thus the nuclear DNA of animals contains many genes coding for proteins that serve essential functions inside the mitochondria; in plants and algae, the nuclear DNA contains many genes specifying proteins required in chloroplasts. In both cases, the DNA sequences of these nuclear genes still show clear evidence of their bacterial origins.
Eukaryotic Genomes Are Big
Natural selection has evidently favored mitochondria with small genomes. By contrast, the nuclear genomes of most eukaryotes seem to have been free to enlarge. Perhaps the eukaryotic way of life has made large size an advantage: predatory cells, for example, typically need to be bigger than their prey, and cell size generally increases in proportion to genome size. Whatever the reason, the genomes of most eukaryotes have become hundreds of times larger than those of bacteria and archaea (Figure 1–30).
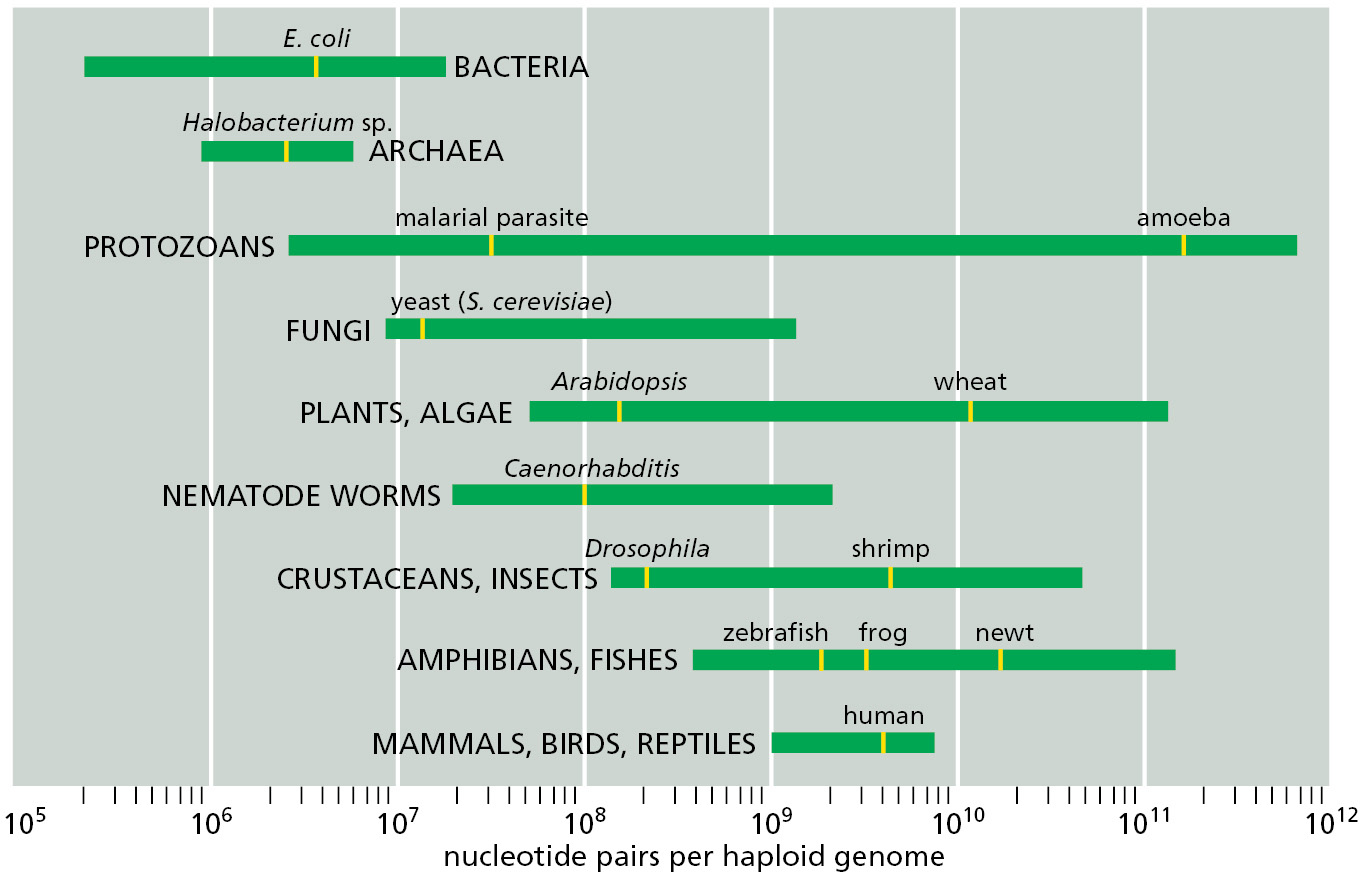
The freedom to be extravagant with DNA has had profound implications. Eukaryotes not only have more genes than prokaryotes; they also have vastly more DNA that does not code for protein or RNA. The human genome contains about 700 times as many nucleotide pairs as the genome of a typical bacterium such as E. coli, but it contains only about 4.5 times as many protein-coding genes because a much greater proportion of the human genome does not code for protein (∼98.5% compared to 11% in E. coli). The estimated genome sizes and gene numbers for a few selected eukaryotes are compared with the bacterium E. coli in Table 1–2; we will discuss shortly how each of these organisms serves as a model organism.
TABLE 1–2 Some Model Organisms and Their Genomes |
||
Organism |
Approximate genome size* (nucleotide pairs) |
Approximate number of protein-coding genes** |
Escherichia coli (bacterium) |
4.6 × 106 |
4300 |
Saccharomyces cerevisiae (yeast) |
12.5 × 106 |
6600 |
Caenorhabditis elegans (roundworm) |
100 × 106 |
20,000 |
Arabidopsis thaliana (plant) |
135 × 106 |
27,000 |
Drosophila melanogaster (fruit fly) |
180 × 106 |
14,000 |
Danio rerio (zebrafish) |
1400 × 106 |
26,000 |
Mus musculus (mouse) |
2800 × 106 |
20,000 |
Homo sapiens (human) |
3100 × 106 |
20,000 |
Eukaryotic Genomes Are Rich in Regulatory DNA
As discussed in Chapter 4, much of our nonprotein-coding DNA is almost certainly dispensable “junk,” retained during evolution like a mass of old papers because, when there is little pressure to keep an archive small, it is easier to retain everything than to sort out the valuable information and discard the rest. Certain exceptional eukaryotic species, such as the puffer fish, bear witness to the profligacy of their relatives; they have somehow managed to rid themselves of large quantities of nonprotein-coding DNA, and, yet, they appear similar in structure, behavior, and fitness to related species that have vastly more such DNA.
Even in compact eukaryotic genomes such as that of the puffer fish, there is more nonprotein-coding DNA than protein-coding DNA. As in all eukaryotic organisms, at least some of the noncoding DNA certainly has important functions. In particular, it regulates the expression of genes. With this regulatory DNA, eukaryotes have evolved distinctive, highly sophisticated ways of controlling when and where a gene is brought into play. Elaborate mechanisms for gene regulation are especially crucial for the formation and function of complex multicellular organisms, which have many different cell types, each with different functions, as we now discuss.
Eukaryotic Genomes Define the Program of Multicellular Development
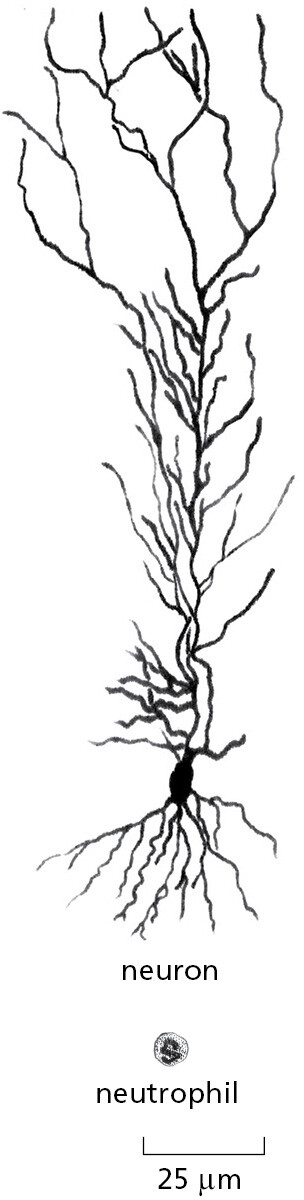
The cells in an individual animal or plant are extraordinarily varied. Blood cells, skin cells, bone cells, nerve cells—they seem as dissimilar as any cells could be (Figure 1–31). Yet all these cell types are the descendants of a single fertilized egg cell, and all (with very minor exceptions) contain identical copies of the genome of the species.
The differences result from the way in which the cells make selective use of their genetic instructions according to their developmental history and the cues they receive from their surroundings in a developing embryo. The DNA is not just a shopping list specifying the molecules that every cell must have, and the cell is not an assembly of all the items on the list. Rather, the cell behaves as a multipurpose machine, with sensors to receive environmental signals and with highly developed abilities to call different sets of genes into action according to signals it receives. The genome in each cell is big enough to accommodate the information that specifies an entire multicellular organism, but in any individual cell only part of that information is used.
Many genes in the eukaryotic genome code for proteins that regulate the activities of other genes, a topic discussed in detail in Chapter 7. Most of these regulatory genes encode transcription regulators that act by binding, directly or indirectly, to the many different DNA sequences that control which genes are to be expressed, and at what levels. Eukaryotic genomes also produce many noncoding RNA molecules; as the name implies, they are not translated into protein but control gene expression in a variety of ways. The expanded genome of eukaryotes therefore not only specifies the hardware of the cell but also stores the software that controls how that hardware is used (Figure 1–32).
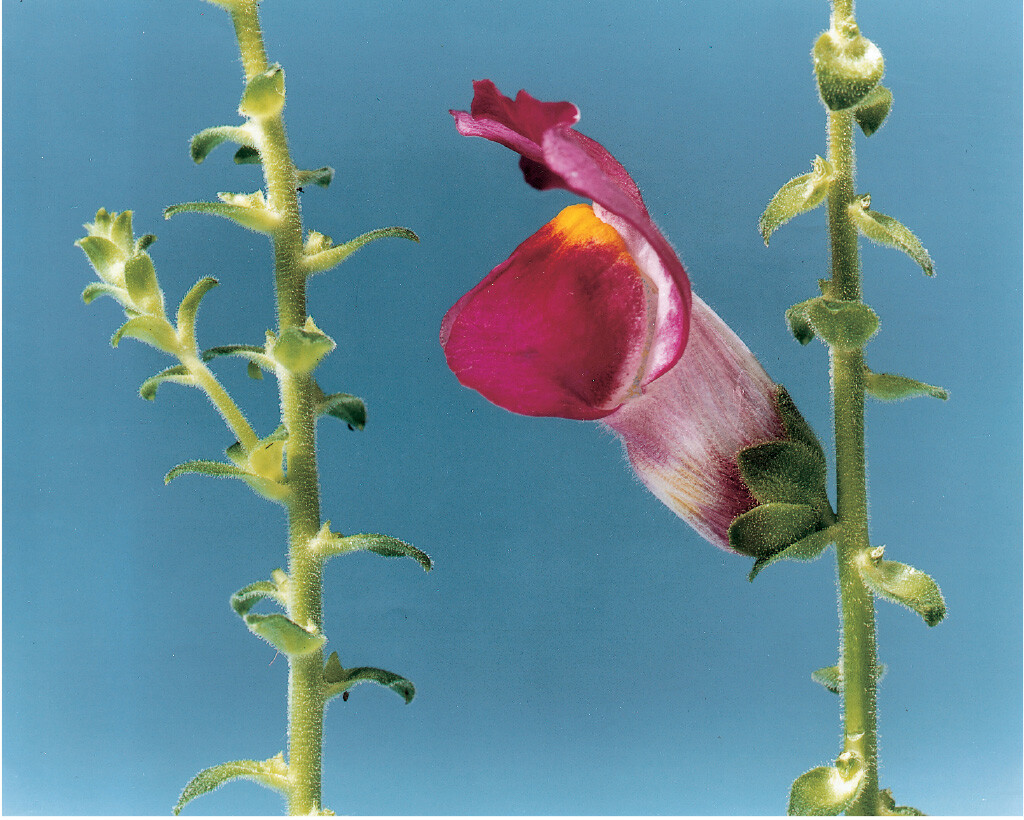
Cells do not just passively receive signals; rather, they actively exchange signals with their neighbors. Thus, in a developing multicellular organism, an internal control system governs each cell that has different consequences depending on the messages exchanged. The outcome, astonishingly, is a precisely patterned array of cells of different types, each displaying a character appropriate to its position in the multicellular structure.
Many Eukaryotes Live as Solitary Cells
Many species of eukaryotic cells lead a solitary life. As we have seen, some of these single-cell organisms are hunters, some are photosynthesizers, still others are scavengers. Figure 1–33 conveys something of the astonishing variety of single-cell eukaryotes, whose anatomy can be remarkably elaborate, including such structures as sensory bristles, photoreceptors, sinuously beating cilia, leglike appendages, mouth parts, stinging darts, and muscle-like contractile bundles. Although they are single cells, they can be as intricate, as versatile, and as complex in their behavior as many multicellular organisms (Figure 1–34, Movie 1.4, and Movie 1.5).
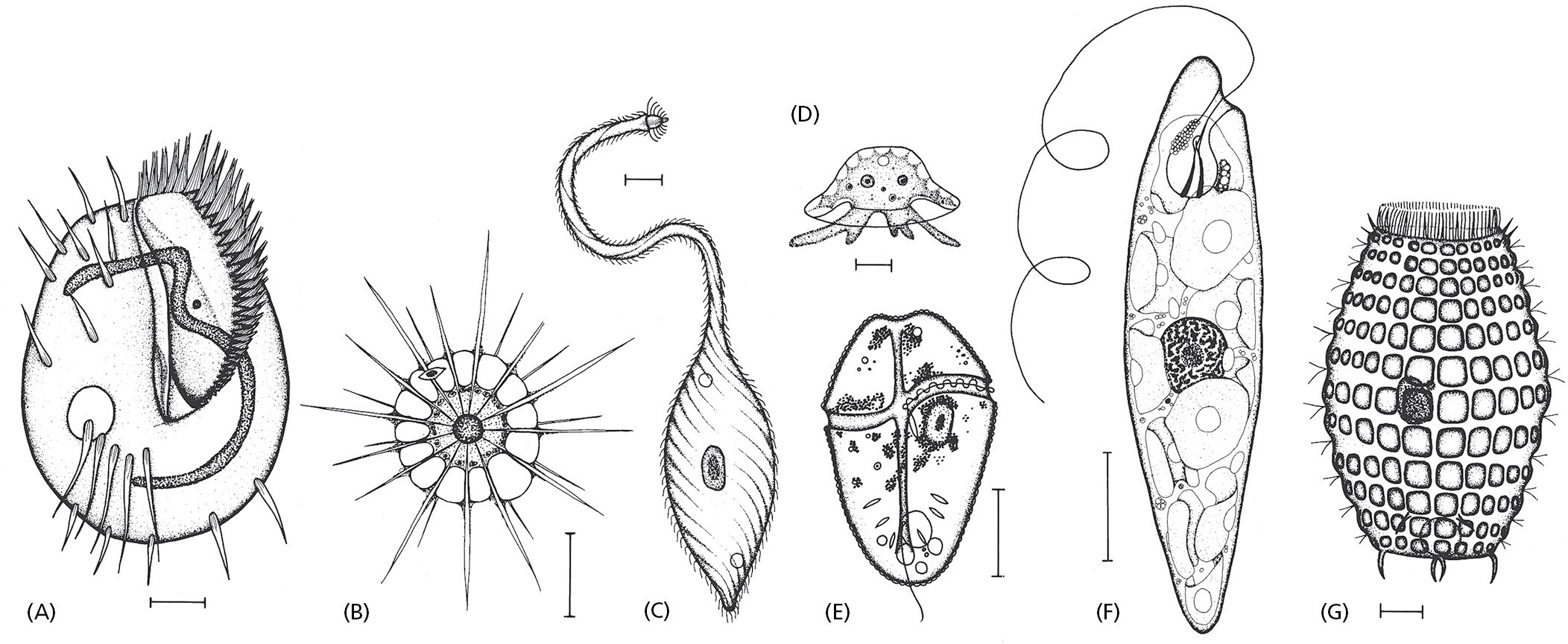
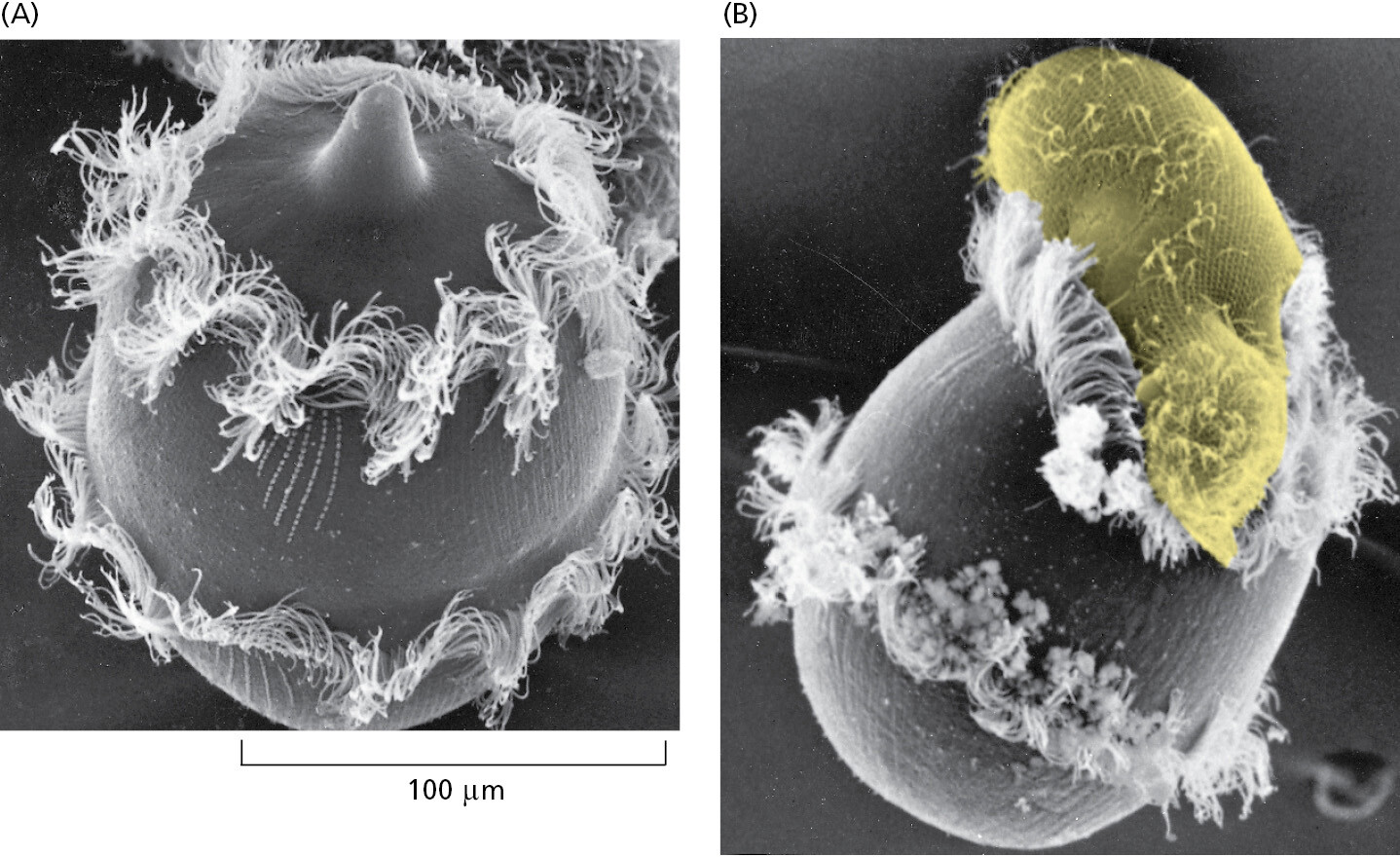
Humans tend to focus on plants and animals, while neglecting single-cell eukaryotes because (along with bacteria and archaea) they are microscopic. But thanks to DNA comparisons, we now know that the genomes of single-cell eukaryotes are far more evolutionarily diverse than those of multicellular animals and plants, meaning that animals and plants arose relatively late in the complex and fascinating eukaryotic pedigree. With genome data, we can position the many different single-celled eukaryotes in the tree of life and identify our closest relatives (Figure 1–35). Scientists are using this information to probe the origins of multicellularity, with a focus on what these strange creatures can tell us about our own evolutionary past.
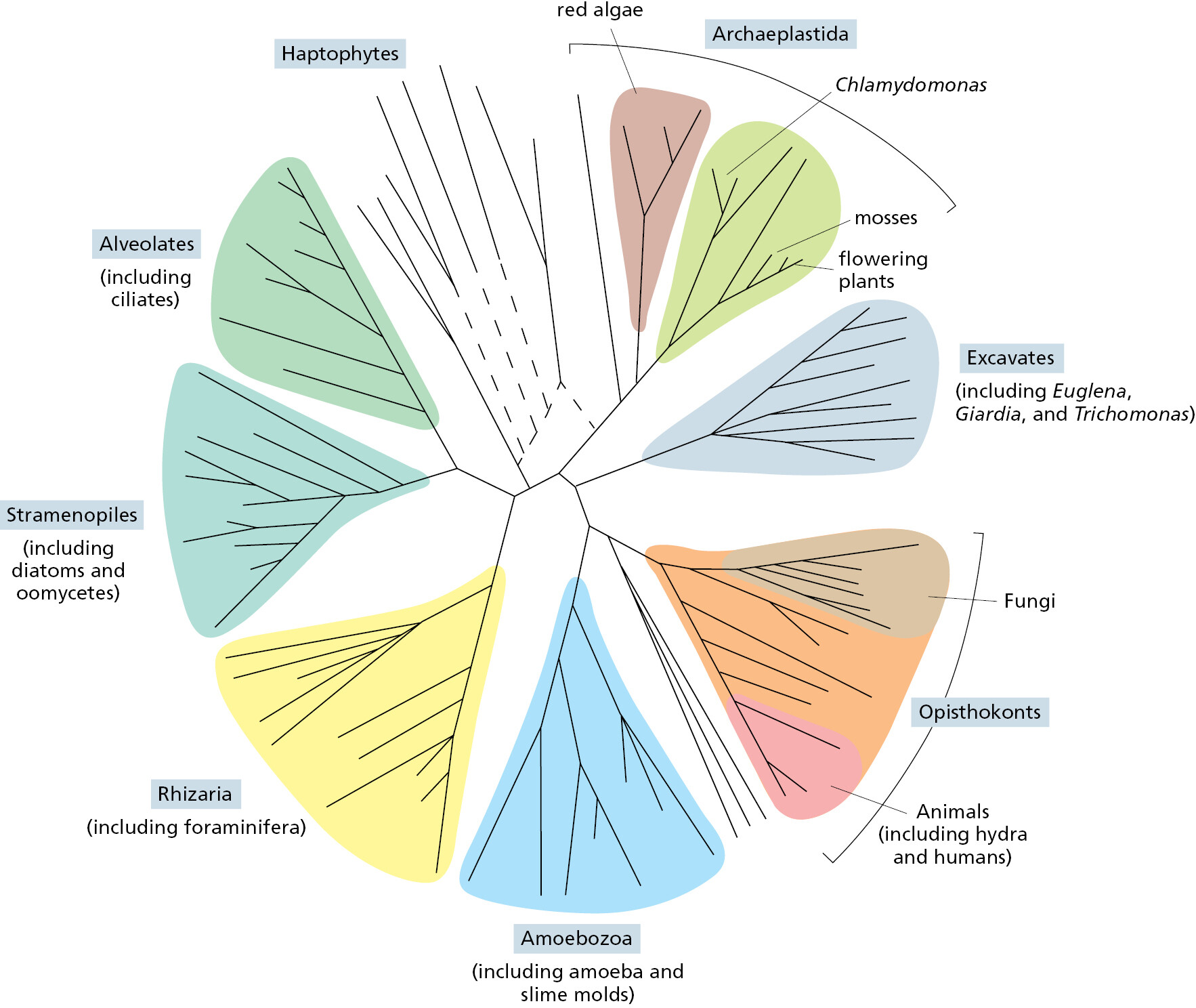
Summary
Eukaryotic cells, by definition, keep most of their DNA in a separate membrane-enclosed compartment—the nucleus. They have, in addition, an elaborate set of other organelles, each carrying out different functions, such as the oxidation of food-derived molecules and production of ATP in mitochondria. Eukaryotic cells also contain a cytoskeleton for structural support and movements. There is compelling evidence that mitochondria and, in plants and algae, chloroplasts evolved from captured symbiotic bacteria, which explains why these organelles contain their own DNA and ribosomes.
Eukaryotic cells typically have 3–8 times as many protein-coding genes as bacteria and archaea and often a thousand times more noncoding DNA. Although much of this DNA is probably unimportant, some of it allows for great complexity in the regulation of gene expression, as required for the construction of complex multicellular organisms containing many different cell types.
Glossary
- organelle
- Subcellular compartment or large macromolecular complex, often but not always membrane-enclosed, that has a distinct structure, composition, and function. Examples of membrane-enclosed organelles are the nucleus, mitochondrion, ER, and Golgi apparatus; examples of organelles that form as biomolecular condensates and lack a membrane are the nucleolus and centrosomes.
- biomolecular condensate
- An aggregate inside cells, formed by a process analogous to liquid–liquid phase separation and based on fluctuating weak interactions between scaffold proteins; concentrates selected protein and RNA molecules in a membraneless compartment.
Endnotes
- Genome size includes an estimate for the amount of highly repeated, noncoding DNA sequence, which does not appear in genome databases. Return to reference *
- There are also genes that code for functional RNA molecules that do not code for proteins. Return to reference **