THE TRANSPORT OF PROTEINS INTO MITOCHONDRIA AND CHLOROPLASTS
Mitochondria and chloroplasts (a specialized form of plastids in green algae and plant cells) are double membrane–enclosed organelles. They specialize in ATP synthesis, using energy derived from electron transport and oxidative phosphorylation in mitochondria and from photosynthesis in chloroplasts (discussed in Chapter 14). Although both organelles contain their own DNA, ribosomes, and other components required for protein synthesis, almost all of their proteins are encoded in the cell nucleus and imported from the cytosol. Each imported protein must reach the particular organelle subcompartment in which it functions.
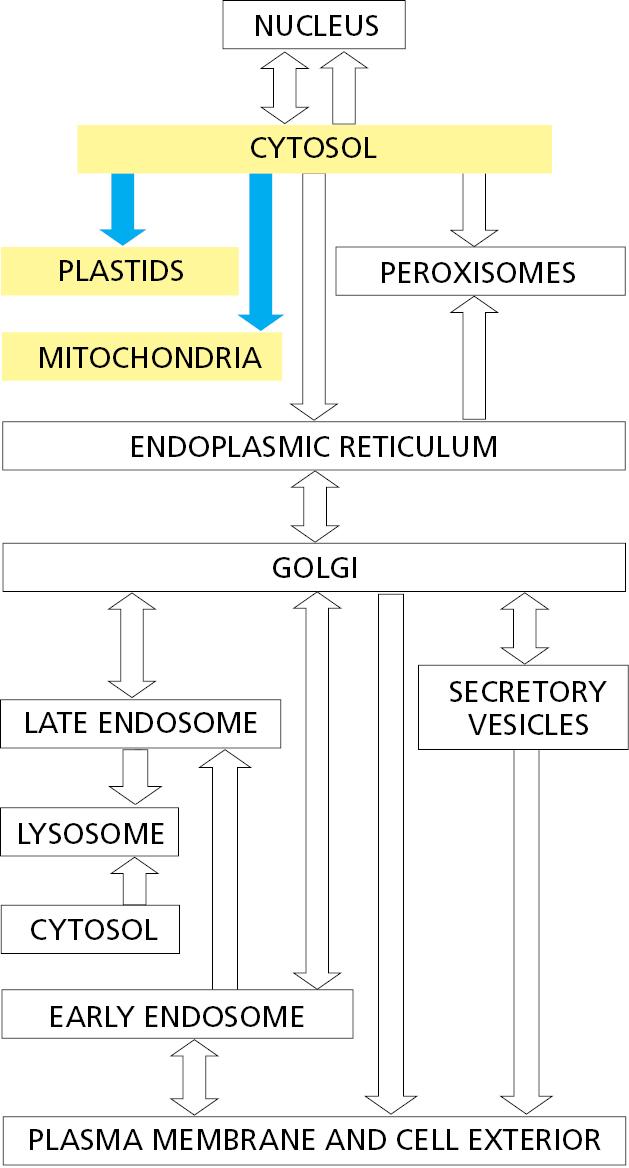
The different subcompartments in mitochondria are formed by the two concentric mitochondrial membranes (Figure 12–47A): the inner mitochondrial membrane, which encloses the matrix space and forms extensive invaginations called cristae, and the outer mitochondrial membrane, which is in contact with the cytosol. The space between the inner and outer membranes is subdivided into the crista space and intermembrane space, with protein complexes at the junctions where the cristae invaginate. Chloroplasts have an outer and inner membrane, which enclose an intermembrane space, and a stroma, which is the chloroplast equivalent of the mitochondrial matrix space (Figure 12–47B). They have an additional subcompartment, the thylakoid space, which is surrounded by the thylakoid membrane. The thylakoid membrane derives from the inner membrane during plastid development and is pinched off to become discontinuous with it. Each of the subcompartments in mitochondria and chloroplasts contains a distinct set of proteins.
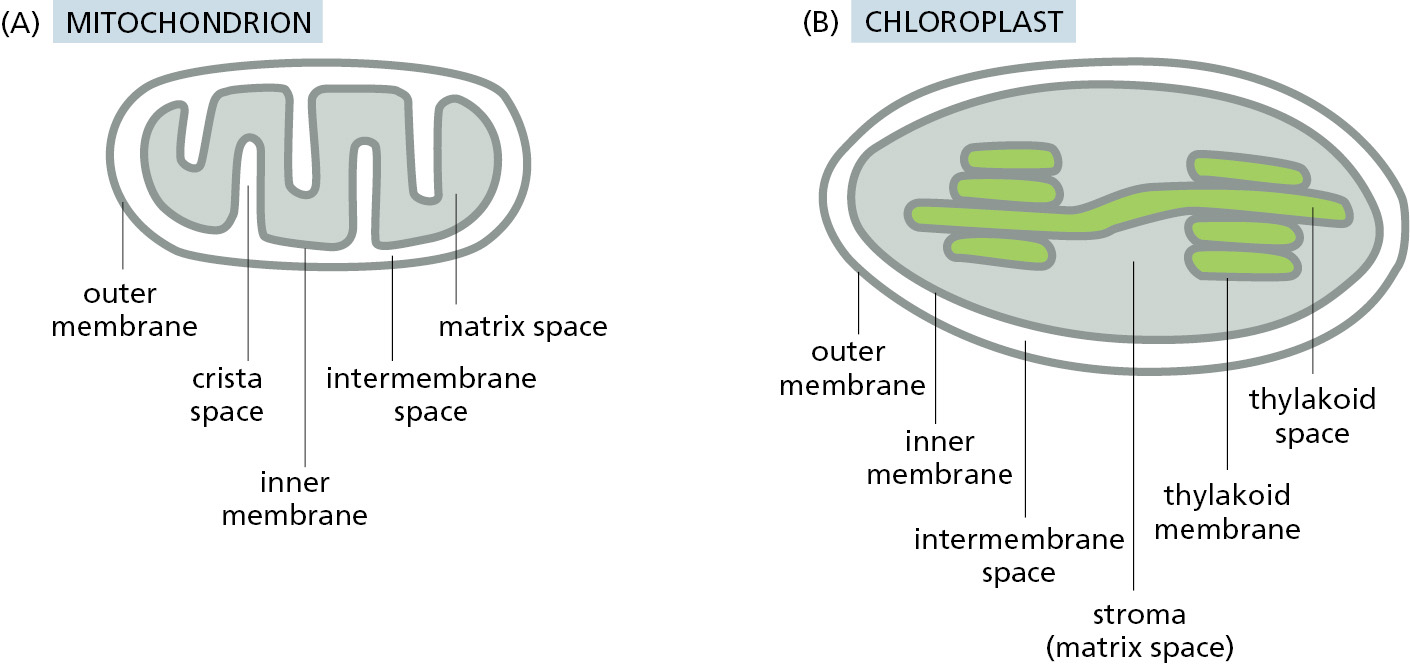
New mitochondria and chloroplasts are produced by the growth of preexisting organelles, followed by fission (discussed in Chapter 14). The growth depends mainly on the import of proteins from the cytosol. Many of the core principles of protein import into mitochondria and chloroplasts are similar to the analogous process of protein import into the ER we discussed earlier. However, the presence of multiple membranes and subcompartments adds to the complexity of delivering newly imported proteins to the correct location. This section explains how it occurs.
Translocation into Mitochondria Depends on Signal Sequences and Protein Translocators
One or more signal sequences direct all mitochondrial precursor proteins to their appropriate mitochondrial subcompartment. Many proteins entering the matrix space contain a signal sequence at their N-terminus that a signal peptidase rapidly removes after import. Other imported proteins, including all outer membrane and many inner membrane and intermembrane-space proteins, have internal signal sequences that are not removed. The signal sequences are both necessary and sufficient for the import and correct localization of the proteins: when genetic engineering techniques are used to link these signals to a cytosolic protein, the signals direct the protein to the correct mitochondrial subcompartment. Thus, the principles of the signal hypothesis, conceived to explain how proteins are segregated to the ER, also apply to mitochondria.
Multisubunit protein complexes that function as protein translocators mediate protein movement across or into mitochondrial membranes (Figure 12–48A). To provide access to each mitochondrial subcompartment, protein translocator complexes are located in both the inner and outer mitochondrial membranes. In general, each translocator has the capacity to recognize particular types of signals and serves as a conduit across or into the membrane within which it resides. Together, these translocators direct ∼1500 different precursor proteins from the cytosol to the appropriate subcompartment of mitochondria: the outer membrane, the intermembrane space and crista space, the inner membrane, and the matrix space.
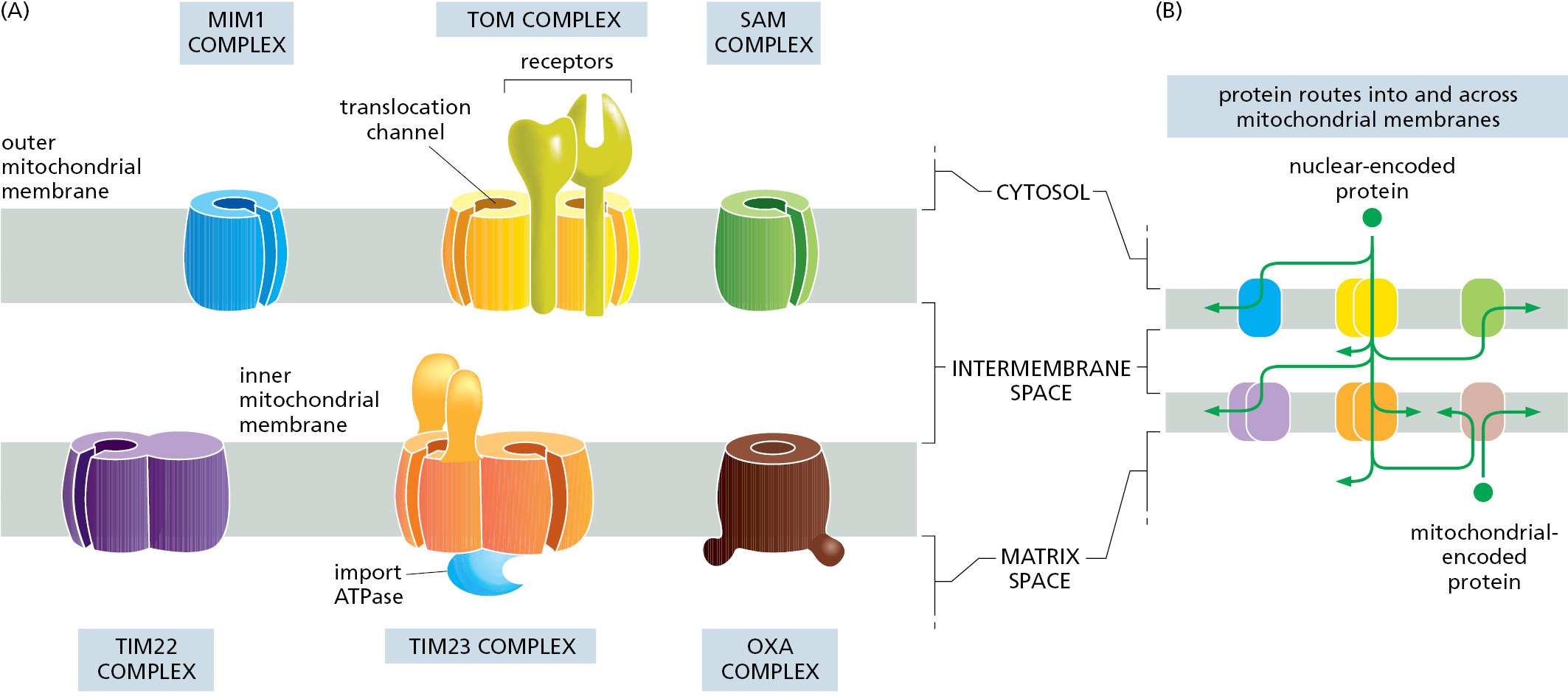
The organization of signals in a precursor protein ultimately controls which translocator(s) the precursor protein engages and the order in which the signals are used to reach the protein’s final destination inside mitochondria. This combinatorial system means that there is sometimes more than one route to reach a particular destination, the same way that different subway lines can take you from Brooklyn to Times Square in New York City. For example, membrane proteins residing in the inner mitochondrial membrane use at least three routes to arrive there. Figure 12–48B shows the possible routes to each mitochondrial subcompartment and the translocator complexes that direct proteins there.
The TOM complex is required for the import of nearly all nucleus-encoded mitochondrial proteins. It initially recognizes their signal sequences and transports them from the cytosol into the intermembrane space. From here, different mitochondrial proteins follow different itineraries depending on sequence features encoded in the protein. β-Barrel proteins, which are particularly abundant in the outer membrane, are passed to the SAM complex for insertion and folding in the outer membrane. Two different TIM complexes mediate protein transport at the inner membrane. Matrix proteins use the TIM23 complex for transport, while inner membrane proteins use the TIM22 complex, the TIM23 complex, or the OXA complex for insertion. The remainder of proteins stay in the intermembrane space where they function.
In addition to the ∼99% of mitochondrial proteins that must be imported from the cytosol, a handful of membrane proteins are encoded by the mitochondrial genome in all eukaryotes. These proteins are synthesized by mitochondrial ribosomes and inserted into the inner membrane by the OXA complex. Mitochondrially encoded membrane proteins are assembled with nuclear-encoded membrane proteins imported from the cytosol to form functional protein complexes such as the respiratory-chain complexes used for energy production (see Chapter 14). How cells communicate between the mitochondria and nucleus to ensure equal expression of the proteins that build inner membrane complexes is not understood.
Mitochondrial Proteins Are Imported Post-translationally as Unfolded Polypeptide Chains
As we learned in an earlier section, protein translocation into the ER usually occurs as the protein is being synthesized by ribosomes that are tightly coupled to the ER protein translocator. The binding of ribosomes to the translocator during protein import is what gives the rough ER its characteristic appearance. In contrast, the protein translocators in the mitochondrial outer membrane do not bind to ribosomes, and most mitochondrial proteins are imported by a post-translational mechanism. This is why very few ribosomes are observed on the surface of mitochondria.
As with ER translocation, mitochondrial protein import can be reconstituted in a cell-free reaction in the test tube. In such experiments, a radioactively labeled mitochondrial precursor protein is mixed with purified mitochondria to permit import into the organelle. By changing the conditions in the test tube, it is possible to establish the biochemical requirements for import, to trap intermediates in the process, and to identify which translocators are used. Most of our knowledge about the molecular mechanism of mitochondrial import comes from analysis in cell-free reactions.
Mitochondrial precursor proteins do not immediately fold into their native structures after they are synthesized; instead, they remain unfolded in the cytosol through interactions with other proteins. Some of these interacting proteins are general chaperones of the hsp70 family (discussed in Chapter 6), whereas others are dedicated to mitochondrial precursor proteins and bind directly to their signal sequences. All the interacting proteins help to prevent the precursor proteins from aggregating or folding up spontaneously before they engage with the TOM complex in the outer mitochondrial membrane. As a first step in the import process, the import receptors of the TOM complex bind the signal sequence of the mitochondrial precursor protein. The unfolded polypeptide chain is then fed—signal sequence first—into the translocation channel within the TOM complex as the cytosolic interacting proteins are stripped off.
Once the translocating protein protrudes into the intermembrane space, sequences within the polypeptide chain determine what happens next. For example, proteins destined for the matrix or inner membrane engage one of the TIM complexes and are either translocated across or inserted into the inner membrane. It is possible to rapidly cool a cell-free mitochondrial import reaction to arrest the proteins at an intermediate step during translocation. Experiments examining an arrested protein destined for the matrix show that it spans both the inner and outer mitochondrial membranes: its N-terminal signal sequence has been removed by the signal peptidase located in the matrix, while the C-terminal part of the protein is still exposed outside the mitochondria. We can therefore conclude that precursor proteins can pass through both mitochondrial membranes at once to enter the matrix space (Figure 12–49).
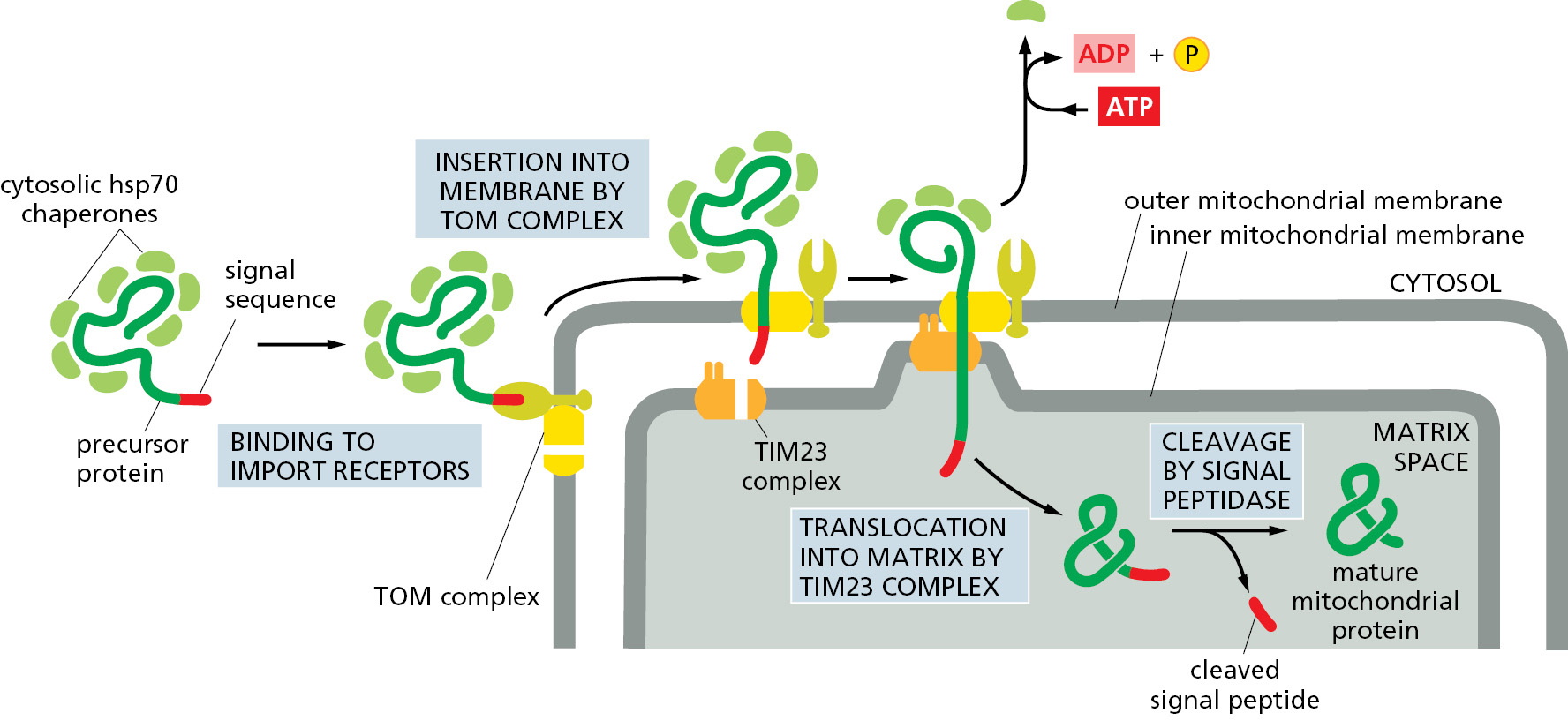
Although the TOM and TIM complexes usually work together to translocate precursor proteins across both membranes at the same time, they are capable of operating independently. In isolated outer membranes, for example, the TOM complex can translocate the signal sequence of precursor proteins across the membrane. Similarly, if the outer membrane is experimentally removed from isolated mitochondria, the exposed TIM23 complex can efficiently import precursor proteins into the matrix space. The experimental uncoupling of ordinarily linked processes allows each step and translocator system to be studied and understood in greater detail.
Protein Import Is Powered by ATP Hydrolysis, a Membrane Potential, and Redox Potential
Directional transport of proteins requires energy (Figure 12–50). Mitochondrial protein import utilizes three different sources of energy at four discrete sites. ATP, a common fuel in most biological systems, is used at two of these sites: outside the mitochondria and inside the matrix. The other two energy sources are contributed by the membrane potential across the inner mitochondrial membrane and the redox potential of the electron-transport chain. Not all mitochondrial precursor proteins need each of these energy sources to arrive at their final destination.
The initial use of energy, needed by most mitochondrial precursor proteins at the initial stage of the translocation process, serves to maintain the polypeptide in an unfolded state prior to import (see Figure 12–49). As discussed in Chapter 6, the chaperones that carry out this task use cycles of ATP binding and hydrolysis to control their interactions with newly synthesized polypeptides. Chaperone interaction is required to prevent premature folding in the cytosol, while chaperone dissociation is needed to permit transport through the TOM complex.
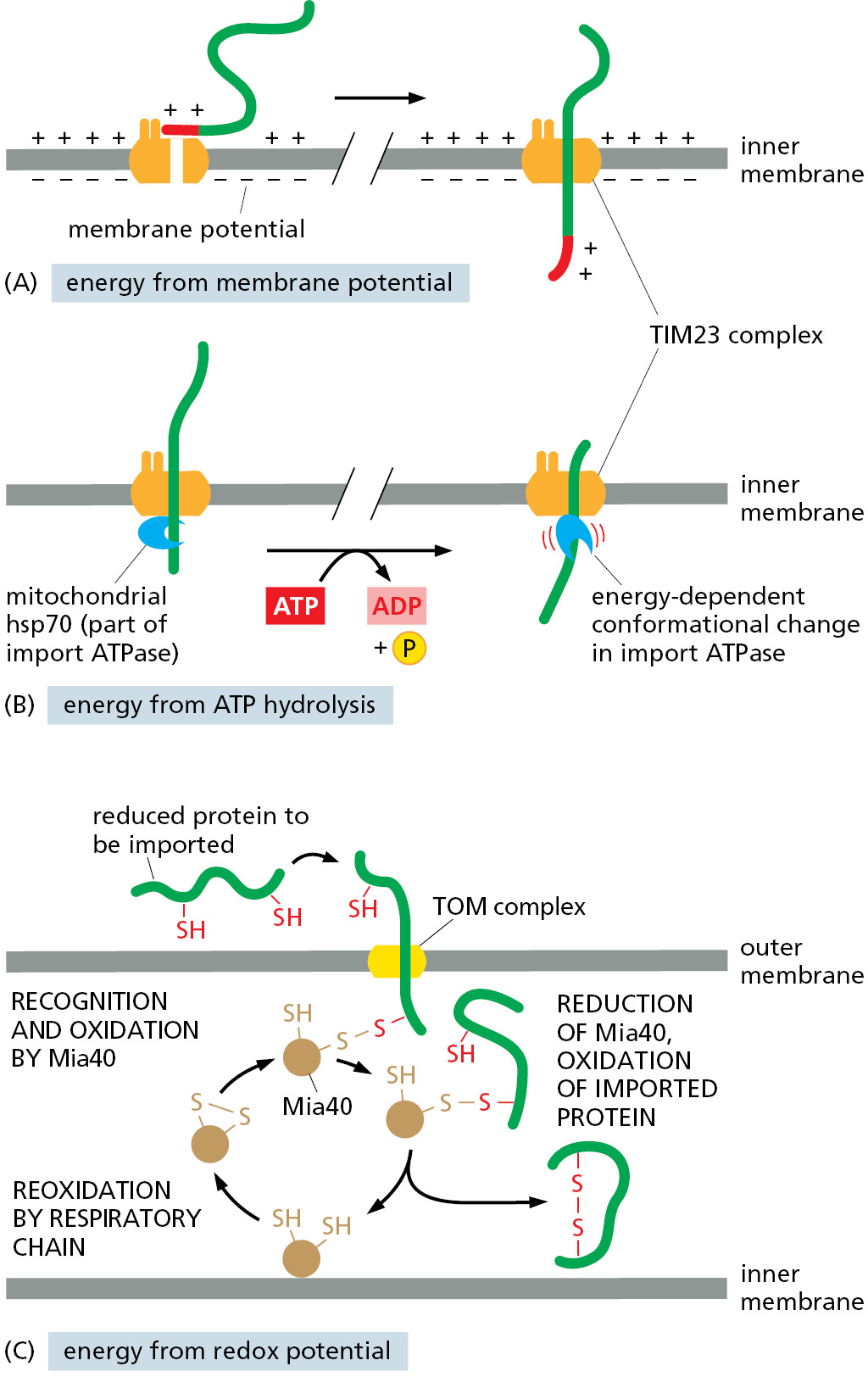
Once the signal sequence has passed through the TOM complex and is bound to a TIM complex, further translocation through the TIM translocation channel requires the membrane potential (Figure 12–50A), which is the electrical component of the electrochemical H+ gradient across the inner membrane (see Figure 11–4). Pumping of H+ from the matrix space to the intermembrane space, driven by electron-transport processes in the inner membrane (discussed in Chapter 14), maintains the electrochemical gradient. The energy in the electrochemical H+ gradient across the inner membrane drives the translocation of the positively charged signal sequences through the TIM complexes by electrophoresis. The same H+ gradient also powers most of the cell’s ATP synthesis by ATP synthase complexes in the inner mitochondrial membrane.
Once the initial segment of a precursor protein reaches the matrix, mitochondrial hsp70 is crucial for completing the import process similar to how BiP is needed for post-translation protein import into the ER. The mitochondrial hsp70 is bound to the matrix side of the TIM23 complex and acts as a motor to pull the precursor protein into the matrix space. Like its cytosolic cousin, mitochondrial hsp70 has a high affinity for unfolded polypeptide chains, and it binds tightly to an imported protein chain as soon as the chain emerges from the TIM translocator in the matrix space. The hsp70 then undergoes an ATP-dependent conformational change that exerts a pulling force on the protein being imported before releasing it. This energy-driven cycle of binding, pulling, and release continues until the protein has completed import through the TIM23 complex (Figure 12–50B). Many imported matrix proteins are passed on to another chaperone protein, mitochondrial hsp60, to assist their folding through cycles of ATP hydrolysis (see Chapter 6).
Certain intermembrane-space proteins that contain cysteine motifs use the difference in redox potential between the cytosol and mitochondria as a source of energy. When a portion of these proteins initially emerges into the intermembrane space, they form a transient covalent disulfide bond to the Mia40 protein (Figure 12–50C). This interaction prevents backsliding of the protein through the TOM complex into the cytosol. The imported proteins are eventually released from Mia40 in an oxidized form containing intrachain disulfide bonds, resulting in a folded protein that is now trapped in the intermembrane space. Mia40 becomes reduced in the process and is then reoxidized by passing electrons to the electron-transport chain in the inner mitochondrial membrane. In this way, the energy stored in the redox potential in the mitochondrial electron-transport chain is tapped to drive protein import.
Transport into the Inner Mitochondrial Membrane Occurs Via Several Routes
The three different translocators in the inner mitochondrial membrane (see Figure 12–48) are all capable of membrane protein insertion. Different subsets of inner mitochondrial membrane proteins take different routes to reach one of these translocators for insertion into the membrane.
In the most common translocation route, a precursor that begins in the cytosol uses the TOM and TIM23 complexes to begin import into the matrix. However, only the N-terminal signal sequence of the transported protein actually enters the matrix space (Figure 12–51A). A hydrophobic amino acid sequence, strategically located after the N-terminal signal sequence, is recognized as a transmembrane domain by the TIM23 complex. This allows insertion of the transmembrane domain into the inner membrane and prevents further translocation into the matrix, perhaps through a lateral gate analogous to that found in the ER-resident Sec61 translocator. The remainder of the protein enters the intermembrane space through the TOM complex, and the signal sequence is cleaved off in the matrix.
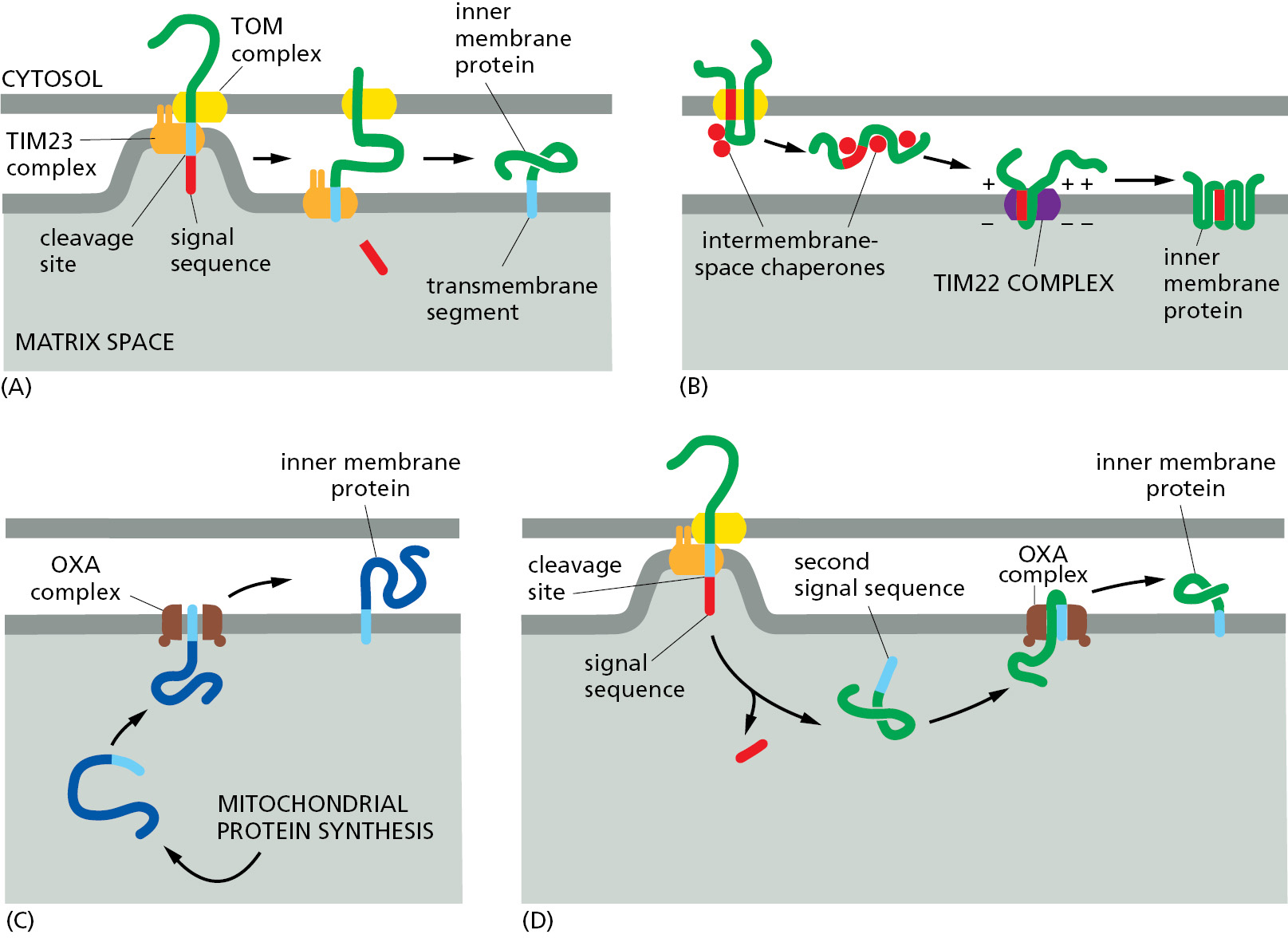
The second transport route to the inner membrane is specialized for a family of metabolite-specific transporters that transfer a vast number of small molecules across the inner membrane. These transporters supply substrates for metabolic enzymes in the mitochondrial matrix, such as those of the citric acid cycle, and export their products back to the cytosol. These multipass transmembrane proteins use internal signal sequences to enter the intermembrane space through the TOM complex. They engage intermembrane-space chaperones that guide them to the TIM22 complex, where hydrophobic transmembrane regions partition into the inner membrane. This insertion process requires the membrane potential to ensure that appropriate regions of the protein are transported to the matrix side so that the transporter acquires the correct topology (Figure 12–51B).
The final insertion route into the inner membrane uses the OXA complex. As mentioned earlier, the OXA complex also inserts the few membrane proteins that are encoded and translated in the mitochondrial matrix (Figure 12–51C). Thus, the OXA complex can only be accessed from the matrix side of the membrane. For this reason, nuclear-encoded membrane proteins that rely on the OXA complex for insertion must first use TIM23 to translocate into the matrix (Figure 12–51D). Here, the N-terminal signal sequence is removed to expose a hydrophobic signal sequence that is then used by the OXA complex for insertion into the inner membrane.
Bacteria and Mitochondria Use Similar Mechanisms to Insert β Barrels into Their Outer Membrane
As discussed earlier in this chapter, mitochondria evolved from an ancestral endosymbiont bacterium inside the primordial eukaryotic cell. The outer mitochondrial membrane is therefore evolutionarily related to the outer membrane of Gram-negative bacteria (see Figure 11–17). Both membranes contain porins, abundant pore-forming β-barrel proteins that are permeable to inorganic ions and metabolites (but not to most proteins). The TOM complex only allows proteins containing hydrophobic α helices to exit laterally and thus cannot integrate porins or other β-barrel proteins into the lipid bilayer. Instead, they are first transported through the TOM complex as unfolded proteins into the intermembrane space. Specialized chaperone proteins in the intermembrane space keep the β-barrel proteins from aggregating (Figure 12–52A) until they are inserted and folded by the SAM complex in the outer membrane.
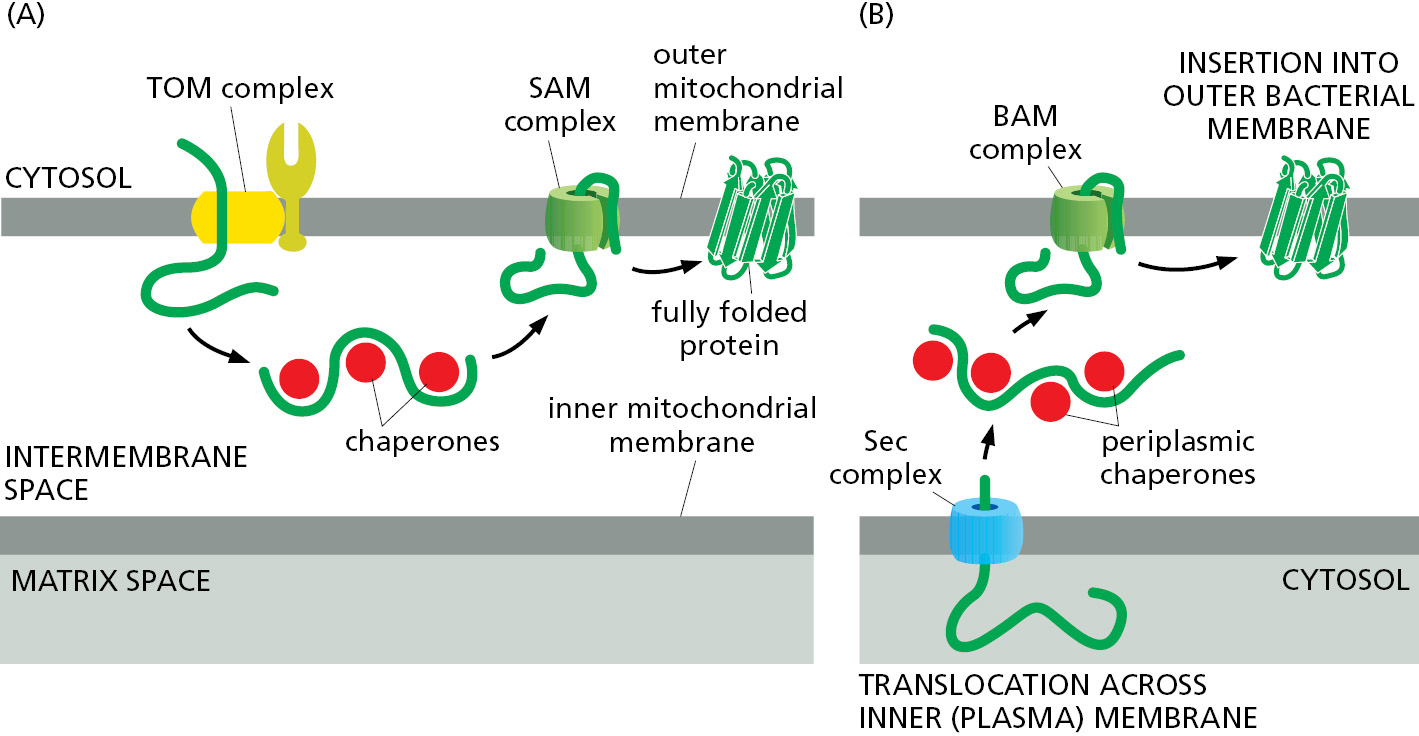
One of the central subunits of the SAM complex is homologous to a bacterial outer membrane protein that helps insert β-barrel proteins into the bacterial outer membrane. In bacteria, β-barrel proteins are inserted from the periplasmic space, which is the topological equivalent of the intermembrane space in mitochondria (Figure 12–52B). This conserved pathway for inserting β-barrel proteins further underscores the endosymbiotic origin of mitochondria. Notably, the central subunits of the TOM and SAM complexes are themselves β-barrel proteins. Thus, preexisting TOM and SAM complexes are required to make more copies of these essential protein translocators.
Two Signal Sequences Direct Proteins to the Thylakoid Membrane in Chloroplasts
Protein transport into chloroplasts resembles transport into mitochondria. Both processes occur post-translationally, use separate translocation complexes in each membrane, require energy, and use multiple types of signal sequences to direct a precursor to the appropriate organelle subcompartment. However, many of the protein components that form the translocation complexes differ. Moreover, whereas mitochondria harness the electrochemical H+ gradient across their inner membrane to drive transport, chloroplasts, which have an electrochemical H+ gradient across their thylakoid membrane but not their inner membrane, use GTP and ATP hydrolysis to power import across their double-membrane envelope. The functional similarities thus result from convergent evolution, reflecting the common requirements for translocation across a double membrane.
Although the signal sequences for import into chloroplasts superficially resemble those for import into mitochondria, a plant cell can have both mitochondria and chloroplasts, so proteins must partition appropriately between the two organelles. Experiments have shown that a cytosolic protein can be directed specifically to a plant cell’s mitochondria if it is experimentally joined to an N-terminal signal sequence of a mitochondrial protein; the same protein joined to an N-terminal signal sequence of a chloroplast protein ends up in chloroplasts. Thus, the import receptors on each organelle distinguish between the different signal sequences.
The same compartments that are found in mitochondria are also in chloroplasts, and each has its distinctive complement of proteins that are selectively delivered there using mechanisms analogous to the mitochondrial systems. However, chloroplasts have an extra membrane-enclosed compartment, the thylakoid. Many chloroplast proteins, including the protein subunits of the photosynthetic system and of the ATP synthase (discussed in Chapter 14), are located in the thylakoid membrane. Many of the components of these vital complexes are encoded in the nuclear genome, and those residing in the thylakoid lumen therefore have to be imported across three membranes. The precursors of these proteins are translocated from the cytosol to their final destination in two steps using bipartite signal sequences. First, they pass across the outer and inner membranes into the stroma guided by an N-terminal chloroplast signal sequence. There, a stromal signal peptidase removes the N-terminal chloroplast signal sequence, unmasking a thylakoid signal sequence that follows it in the sequence of the precursor protein. The thylakoid signal sequence initiates integration into the thylakoid membrane or translocation into the thylakoid space (Figure 12–53A).
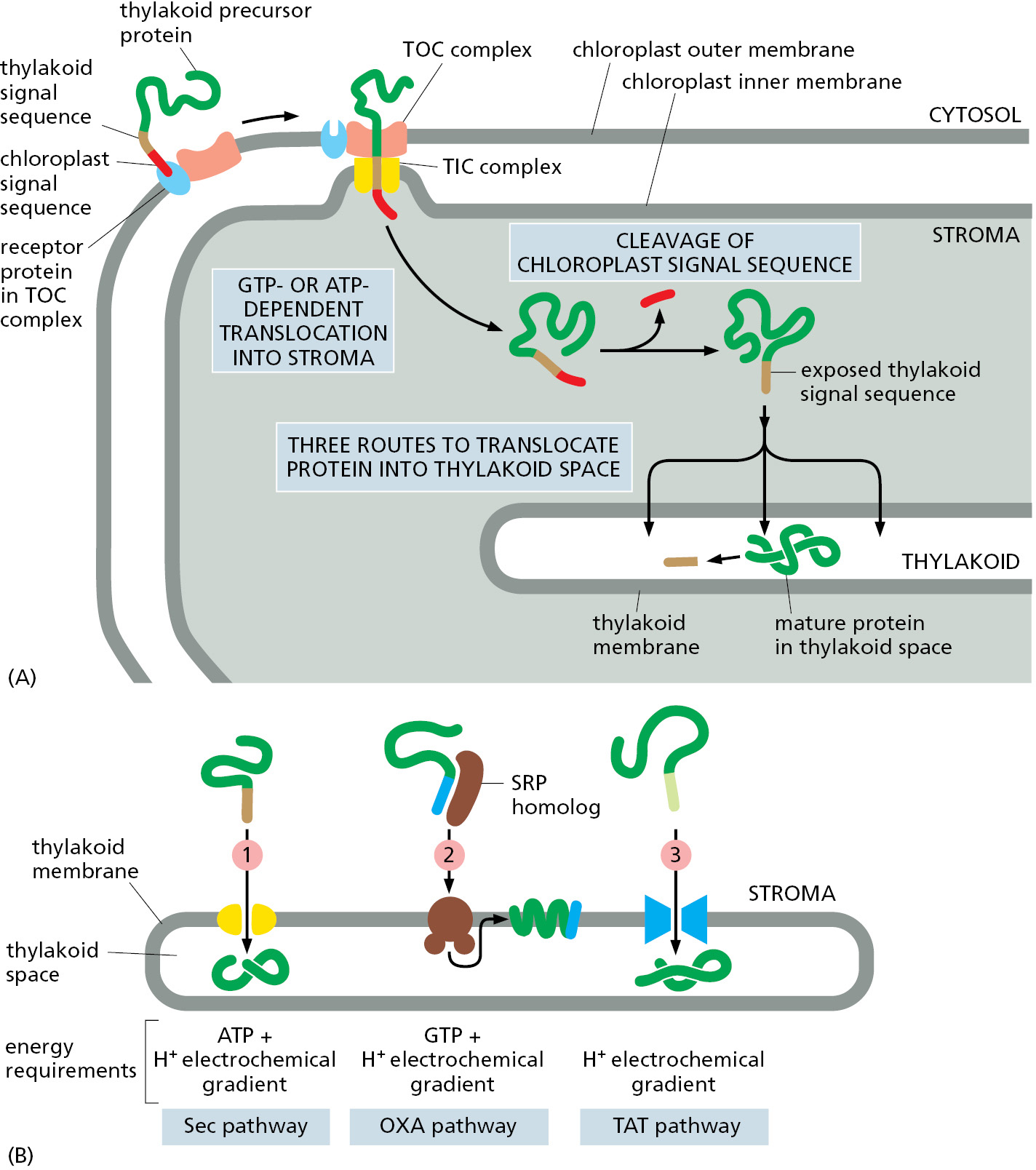
There are three different protein translocators in the thylakoid membrane, each of which recognizes a different type of signal sequence, handles a different subset of thylakoid precursors, and uses energy in different ways (Figure 12–53B). As we saw earlier, the thylakoid membrane is developmentally derived from the inner chloroplast membrane, which is evolutionarily related to the bacterial inner membrane. It is therefore not surprising that each of the three translocators in the thylakoid membrane has homologs that are used for translocation or membrane insertion in bacteria.
Summary
Although mitochondria and chloroplasts have their own genetic systems, they produce less than 1% of their own proteins. Instead, the two organelles import most of their proteins from the cytosol, using similar mechanisms. In both cases, multiple protein translocator complexes in the outer and inner membranes recognize different types of signal sequences to direct a precursor to the correct organelle subcompartment. Proteins are transported in an unfolded state by a post-translational mechanism. Chaperone proteins of the cytosolic hsp70 family maintain the precursor proteins in an unfolded state prior to translocation, and a second set of hsp70 proteins in the matrix space or stroma pulls the polypeptide chain across the inner membrane. Translocation into mitochondria is powered by ATP hydrolysis, a membrane potential across the inner membrane, and the redox potential of the electron-transport chain. Translocation into chloroplasts is powered by GTP and ATP hydrolysis and a membrane potential across the thylakoid membrane. In chloroplasts, import from the stroma into the thylakoid can occur by several routes, distinguished by the protein translocator complex and energy source used.
Glossary
- mitochondria (plural mitochondria)
- Membrane-bounded organelle, about the size of a bacterium, that carries out oxidative phosphorylation and produces most of the ATP in eukaryotic cells.
- inner mitochondrial membrane
- Mitochondrial membrane that encloses the matrix space and forms extensive invaginations called cristae.
- matrix space
- The large internal compartment of the mitochondrion.
- outer mitochondrial membrane
- The mitochondrial membrane that separates the organelle from the cytosol; surrounds the inner mitochondrial membrane.
- intermembrane space
- The compartment in a mitochondrion between the outer and inner mitochondrial membranes.
- TOM complex
- Multisubunit protein complex that transports proteins across the mitochondrial outer membrane.
- SAM complex
- Protein translocator that helps β-barrel proteins to fold properly in the outer mitochondrial membrane.
- TIM complexes
- Protein translocators in the mitochondrial inner membrane. The TIM23 complex mediates the transport of proteins into the matrix and the insertion of some proteins into the inner membrane; the TIM22 complex mediates the insertion of a subgroup of proteins into the inner membrane.
- OXA complex
- Protein translocator in the inner mitochondrial membrane that mediates insertion of inner membrane proteins.
- mitochondrial hsp70
- Part of a multisubunit protein assembly bound to the matrix side of the TIM23 complex that acts as a motor to pull mitochondrial precursor proteins into the matrix space.
- porins
- Channel-forming proteins of the outer membranes of bacteria, mitochondria, and chloroplasts.
- chloroplasts
- Membrane-bounded organelle in green algae and plants that contains chlorophyll and carries out photosynthesis.
- thylakoid
- Flattened sac of membrane inside a chloroplast that contains chlorophyll and other pigments and carries out the light-trapping reactions of photosynthesis. Stacks of thylakoids form the grana of chloroplasts.
- stroma
- (1) “Bedding”: the connective tissue in which a glandular or other epithelium is embedded. Stromal cells provide the environment necessary for the development of other cells within the tissue. (2) The large interior space of a chloroplast, containing enzymes that incorporate CO2 into sugars.