Replisome Movement Within a Dividing Cell
3.4 Bacterial Cell Division
How does a growing bacterial cell divide, or fission, into daughter cells? Bacterial cell fission requires highly coordinated growth and formation of all the cell’s parts. Unlike eukaryotes, prokaryotes synthesize RNA and proteins continually while the cell’s DNA undergoes replication. Bacterial DNA replication is coordinated with the expansion of the cell wall and the separation of the cell into two daughter cells. Bacterial DNA replication is outlined here as it relates to cell division; the genetic aspects of DNA replication are discussed in Chapter 7.
Note: Bacteria do not undergo mitosis or meiosis. These eukaryotic processes are reviewed in eAppendix 2.
Cell Division by Septation
In rod-shaped cells, the envelope elongates to a consistent length by extension of peptidoglycan chains in tracks around the cell, as we saw in Figure 3.13. But as DNA synthesis terminates, the cell divides by a process called septation, the formation of the septum, the partition that divides the envelope. How does the septum actually form, completing two entire cell envelope layers back-to-back? The laboratories of Ethan Garner at Harvard University and of Yves Brun at Indiana University revealed the progression and timing of septum growth (Fig. 3.24).
The septum grows inward from all around the cell equator. As it grows inward, its inner hole constricts and seals off the two daughter cells. The inward growth of peptidoglycan can be seen in Figure 3.24A, where a cell of Bacillus subtilis incorporates d-alanine fluorophores. d-Alanine is an amino acid that gets incorporated into peptidoglycan cross-bridges (Fig. 3.12) but not into proteins, which use only l-form amino acids. Thus, the d-alanine fluorophore labels only peptidoglycan.
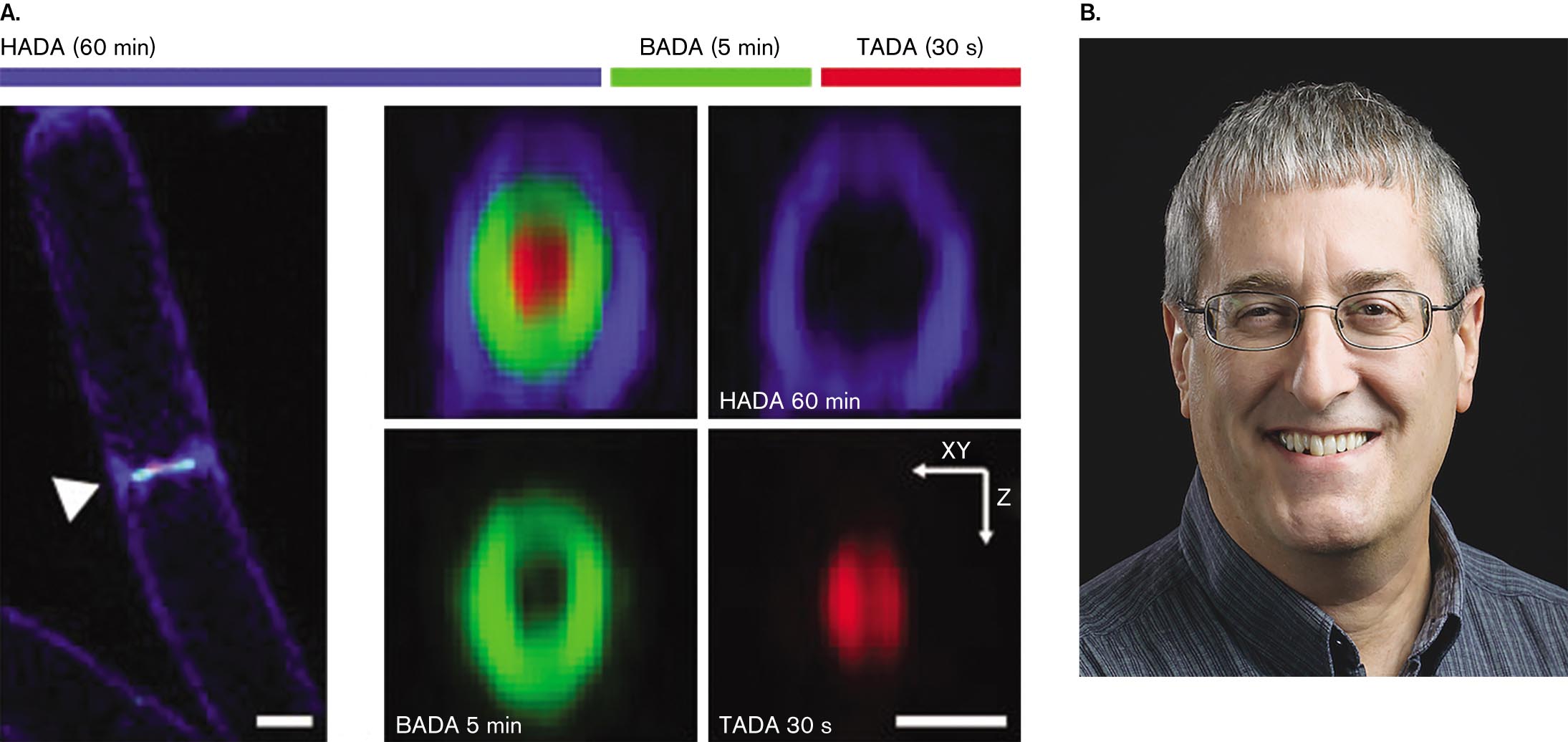
More information
Pulse labeling of several molecules in Bacillus subtilis and a photograph of Yves Brun.
Pulse labeling of several molecules in Bacillus subtilis. The H A D A stage in blue color for 60 minutes, the B A D A stage in green color for 5 minutes, and the T A D A stage in red color for 30 seconds. The first image shows a rod-shaped bacteria and an arrow pointing its center where a brightly colored band is visible. The bacteria is about 4 micrometers long and 0.5 micrometer wide. The second image shows a roughly round shape of a red color region surrounded by a green ring followed by a blue ring. The third image shows H A D A 60 minutes with a blue color ring. The fourth image shows B A D A 5 minutes with a small ring in green color. The fifth image shows T A D A 30 seconds with a round shape in red color, with x-y-plane in horizontal to left direction and z-plane in the vertically downward direction.
A photograph of Yves Brun smiling at the camera. He has gray hair and blue eyes. He is wearing glasses.
Source: Alexandre Bisson-Filho et al. 2017. Science 355:739, fig. 1A.
ALEXANDRE BISSON-FILHO ET AL. 2017 SCIENCE 355:739, FIG. 1APHOTO BY SANDEE MILHOUSEIn the experiment, the d-alanine fluorophores emit one of three different colors, in successive periods of pulse labeling. The initial, longest period (60 minutes) is required to grow the outermost ring of cell wall pinching in; then shorter pulses (5 minutes, 30 seconds) rapidly complete the septum. Garner’s team showed that FtsZ subunit assembly circles around the septum in a “treadmilling” pattern, stepwise around the cell, that directs septal growth.
Septation requires rapid biosynthesis of all envelope components, including membranes and cell wall (Fig. 3.25). Envelope expansion must coordinate the extension of all layers—and regulate the placement and timing of the septum. As we saw earlier (Fig. 3.13), the enzymes of cell wall biosynthesis must coordinate the formation of new links as closely as possible with subunit insertion. The site of septation is uniquely vulnerable because two new large enclosures must form simultaneously. Thus, the enzymes of septum biosynthesis are of great interest as antibiotic targets.
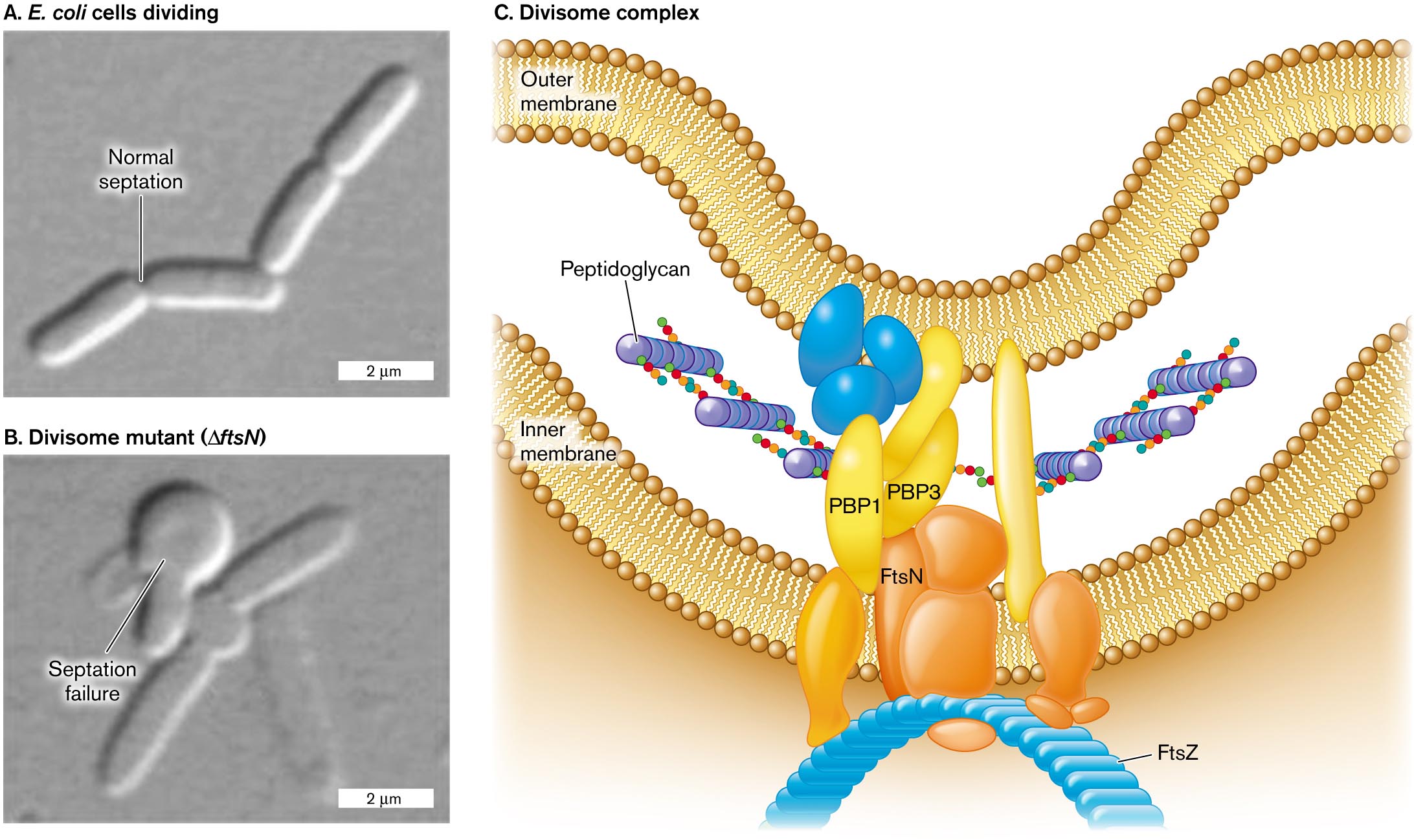
More information
Two micrographs of cell division and an illustration of the divisome complex.
A micrograph of E. coli cells dividing with normal septation. Four rod shaped cells in a chain are visible, each about 3 micrometers long and 0.5 micrometer wide. A split between the first two cells is labeled normal septation.
A micrograph of E. coli septation failure in a divisome mutant. A chain of a few rod shaped cells is visible, with a twisted clump of cells at the center of the chain. The clump is labeled septation failure. The divisome mutant is labeled delta f t s N.
An illustration of the structure of the divisome complex. The complex is located between the inner and outer membrane. Peptidoglycan synthesis is visible, occurring between P B P 1 and P B P 3. The divisome complex consists of several irregularly shaped proteins. F t s N is identified within the complex, and at the base of the complex where the cell shape can be seen curving, F t s Z is identified.
Source: Part C modified from C. Typas et al. 2012. Nat. Rev. Microbiol. 10:123, fig. 2.
B. LIU ET AL. 2015. MOL. MICROBIOL. 95:945B. LIU ET AL. 2015. MOL. MICROBIOL. 95:945The overall process of septation is managed by a protein complex called the divisome (Fig. 3.25C). The divisome manages assembly of the septum with its two envelopes back-to-back. One component of the divisome is FtsZ, which polymerizes to form the Z-ring, as seen previously, in Figure 3.23. Mutations in a gene such as ftsZ cause Escherichia coli to form long filaments instead of dividing normally. Another divisome component, FtsN, helps regulate the timing of constriction of the septum. A cell was constructed that requires an inducer molecule to express FtsN (Fig. 3.25C). Cells that lack FtsN fail to constrict their septum, and their membranes balloon out at cell division. Such divisome components could be targets for new antibiotics, just as penicillin-binding proteins are targets for penicillin.
The bacterial process of cell fission must solve a key problem: how to coordinate septation with the replication of DNA. Indeed, mutations exist that lead to septum formation across DNA with replication incomplete. The result is to “guillotine” the cell. To avoid this disastrous situation, septation is coordinated with DNA replication.
DNA Is Organized in the Nucleoid
The genetic functions of microbial DNA are discussed in detail in Chapters 7–12. Here we focus on the physical organization of DNA within the nucleoid of bacterial and archaeal cells.
Bacteria organize their DNA very differently from eukaryotes. For example, Figure 3.26 shows enteropathogenic E. coli cells growing on a cultured human cell. Enteropathogenic E. coli (EPEC) are diarrheal pathogens that attach to the host cell membrane and inject toxins (discussed in Chapter 25). In this thin-section transmission electron micrograph, each bacterium contains a filamentous nucleoid region that extends through the cytoplasm. In contrast, the nucleus of the eukaryotic cell (not shown) is many times larger than the entire bacterial cell, and the chromosomes it contains are separated from the cytoplasm by the nuclear membrane.
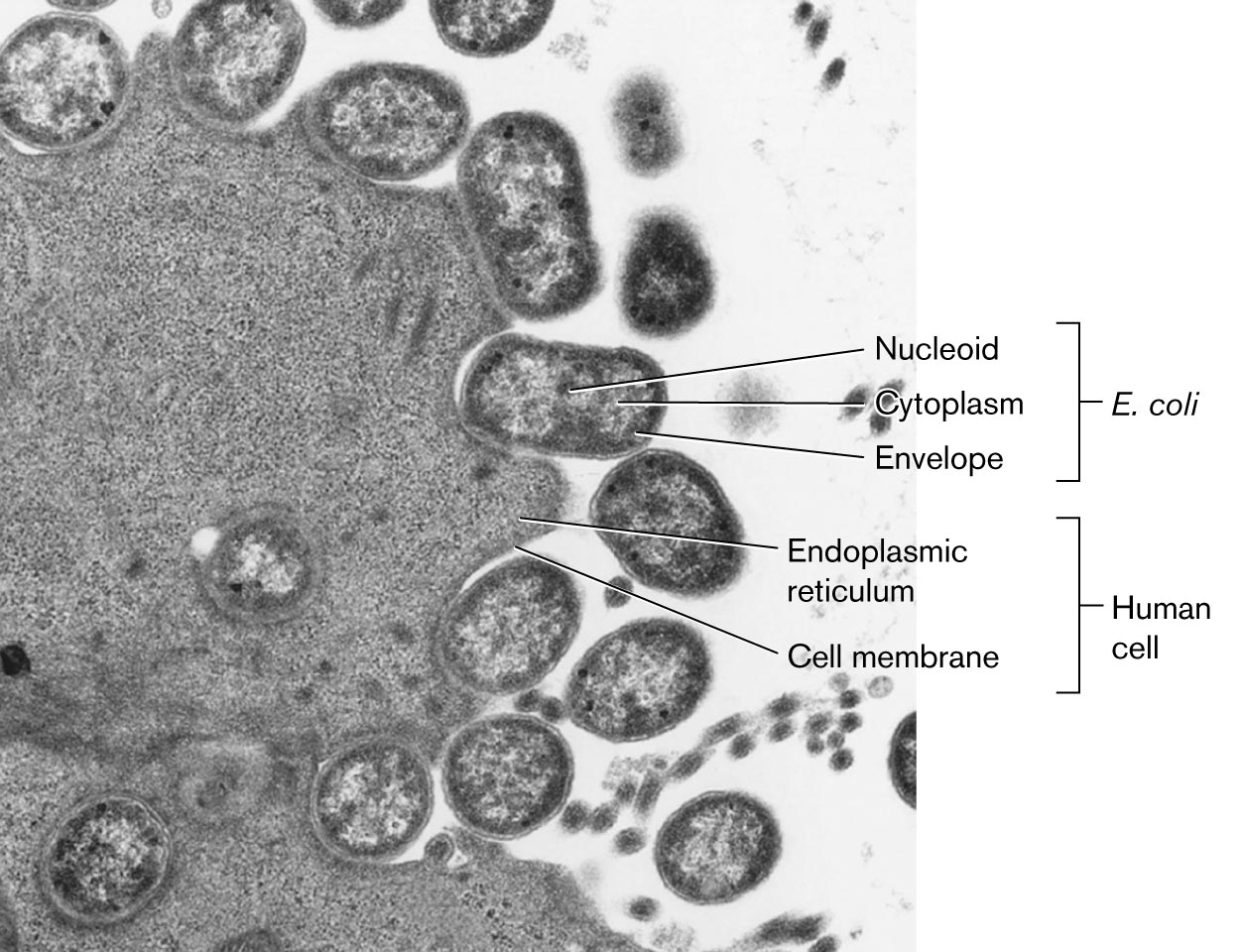
More information
A microscopic image shows bacteria invading a human cell. The cell membrane and the endoplasmic reticulum in the human cells are labeled. Round shaped bacteria are shown closer to the human cell membrane. In the bacterial cells, the nucleoid at the center, the envelope is the outer membrane, and the cytoplasm is between the nucleoid and the envelope The bacteria are identified as E. coli.
Note: In bacteria and archaea, the genome typically consists of a single circular chromosome, but some species have a linear chromosome or multiple chromosomes. In this chapter we focus on the simple case of a single circular chromosome.
In a bacterial cell, the DNA is organized in loops called domains, which extend throughout the cytoplasm. The midpoint on the DNA is the origin of replication, which is attached to the cell envelope at a point on the cell’s equator, halfway between the two poles (Fig. 3.27). To initiate DNA replication, the DNA double helix at the origin is opened by binding proteins, and then DNA polymerase synthesizes new strands in both directions (bidirectionally). The origin and other aspects of DNA replication are covered in detail in Chapter 7.
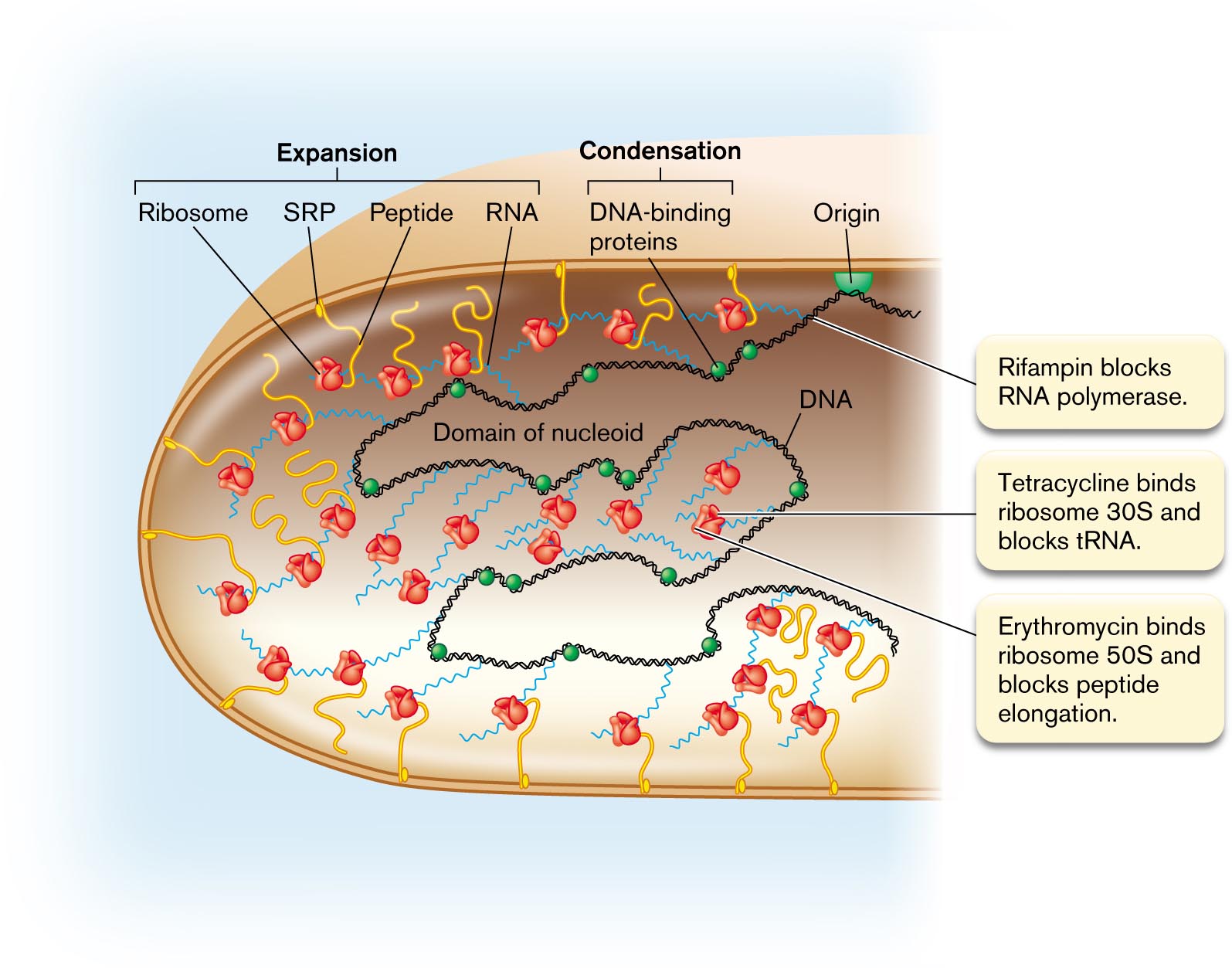
More information
An illustration shows the transcription of D N A and the translation of R N A to peptides. The structure of the domain of nucleoid consists of a chain in the center labeled as D N A. Several spheres bonded on the D N A are labeled as D N A-binding proteins. The D N A is attached with several chains labeled as R N A. Several short curves attached to the outer layer of the nucleoid are labeled as peptides, in which the flower-shaped image is attached at the top end is labeled as the ribosome, and the bottom end is labeled as signal recognition particle. A semi-circle attached to the inner layer is labeled as to origin. The ribosome, signal recognition particle, peptide, and R N A are labeled as expansion. The D N A-binding proteins are labeled as condensation. An annotation pointing toward the D N A reads Rifampin blocks R N A polymerase. Another annotation pointing toward the ribosome reads Tetracycline binds ribosome 30 S and blocks t R N A and Erythromycin binds ribosome 50S and blocks peptide elongation.
How does all of the cell’s DNA fit neatly into the nucleoid? In some bacteria, the domains loop back to the center of the cell, near the origin of replication. Within the domains, the DNA is compacted by supercoils. Supercoils (or superhelical turns) are extra twists in the chromosome, beyond those inherent in the structure of the DNA double helix (discussed in Chapter 7). In most bacteria and in eukaryotes, the extra twists actually go against the twist direction of the DNA helix; thus they tend to unwind DNA slightly.
The supercoiling causes portions of DNA to double back and twist upon themselves, resulting in compaction of the chromosome. Supercoils are generated by enzymes such as gyrase, which are a major target for antibiotics such as quinolones. DNA is also compacted by DNA-binding proteins (green spheres in Fig. 3.27). Binding proteins can respond to the state of the cell; for example, under starvation conditions, when most RNA synthesis ceases, the binding protein Dps is used to organize the DNA into a protected crystalline structure. Such “biocrystallization” by Dps and related proteins may be a key to the extraordinary ability of microbes to remain viable for long periods in stationary phase or as endospores.
Note: In biology, the word “domain” is used in several different ways, each referring to a defined portion of a larger entity.
- DNA domains of the nucleoid are distinct loops of DNA that extend from the origin.
- Protein domains are distinct functional or structural regions of a protein.
- Lipid domains are patches of membrane that are enriched for certain lipids.
- Taxonomic domains are genetically distinct classes of organisms, such as Bacteria, Archaea, and Eukarya.
Transcription and translation are coupled (discussed in Chapter 8). The information encoded in DNA is “read” by the processes of transcription and translation to yield gene products. In bacteria and archaea, some translation is tightly coupled to transcription; the ribosomes bind to mRNA and begin translation even before the mRNA strand is complete. Thus, a growing bacterial cell is full of mRNA strands dotted with ribosomes (Fig. 3.27). Some of the mRNA strands with their growing peptide chains extend to the membrane for protein insertion and secretion.
In rapidly growing bacteria, the DNA is transcribed and the messenger RNA is translated to proteins while the DNA itself is being replicated. This remarkable coordination of replication, transcription, and translation explains why some bacterial cells can divide in as little as 10 minutes. An example is the hot-spring bacterium Geobacillus stearothermophilus cultured at 60°C. DNA synthesis, transcription to RNA, and translation to proteins are discussed further in Chapters 7 and 8.
Some of the newly translated proteins are destined for the cell membrane or for secretion outside. Proteins destined for the membrane are synthesized in association with the membrane, directed there by signal recognition particles (Fig. 3.27). This coupling of transcription and translation to membrane insertion has the effect of expanding the nucleoid into distal parts of the cell, partly counteracting the condensation of DNA by DNA-binding proteins. Membrane protein maturation and secretion are discussed in Chapter 8.
DNA Replication Regulates Cell Division
The process of synthesizing daughter cells begins at the origin of replication, a unique DNA sequence in the chromosome. In bacteria, the origin is attached to a site on the envelope—most commonly, at a point on the cell’s equator (Fig. 3.28 ). At the origin sequence, the DNA double helix begins to unzip, forming two replication forks. At each replication fork, DNA is synthesized by DNA polymerase. The complex of DNA polymerase with its accessory components is called a replisome. The replisome actually has a double complex of DNA polymerase that simultaneously replicates the “leading strand” and the “lagging strand” of the helix. The lag time is short compared with the overall time of replication; thus, as the replisome travels along the DNA, it converts one helix into two progeny helices almost simultaneously (details presented in Chapter 7).
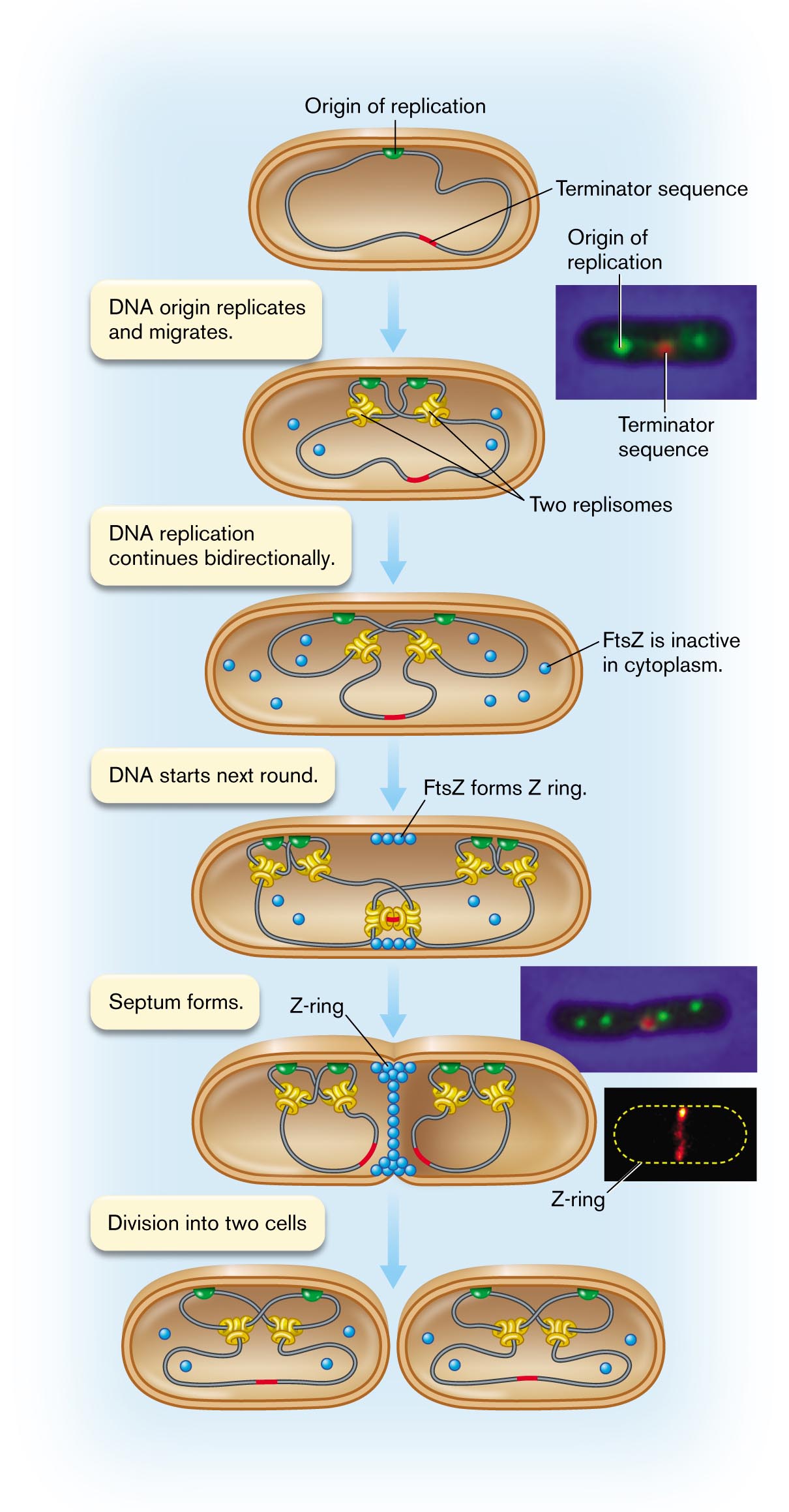
More information
An illustration shows the movement of replisome within a dividing cell. The process begins with the origin of replication and terminator sequence in a cell. An inset shows a microscopic view of the origin of replication and terminator sequence. A note reads as follows: D N A origin replicates and migrates. It leads to the structure of the microbial cell, and there are two replisomes. The note reads D N A replication continues bidirectionally, which leads to the structure in which the cell has become wider that contains several dots labeled as FtsZ is inactive in cytoplasm. The corresponding note reads D N A starts next round that consists of four dots bonded closely in the top of the inner layer is labeled as F t s Z forms Z ring. The note reads a septum forms. An inset shows a microscopic view of the origin of replication sites and the terminator sequence of the splitting cell labeled as Z-ring. The last step shows the division of two cells. Each cell contains two replisomes, two origins of replication sites, and one terminator sequence.
A micrograph showing a fluorescently labeled origin of replication and terminator sequence within a cell. The cell is rod shaped. The origin of replication fluoresces green and the terminator sequence fluoresces red.
A micrograph showing septum formation within a cell. A long rod shaped cell is visible, with a pinched section in the center. Four replisomes fluoresce green and are settled in pairs at either side of the pinch. A terminator sequence fluoresces red.
An image of a pulse labeled Z ring. The shape of a rod-shaped bacterium is loosely identified. A fluorescent band is visible in the center of the shape.
Source: Top 2 insets: Ivy Lau et al. 2003. Mol. Microbiol. 49:731. Bottom inset: Jackson Buss et al. 2015. PLoS Genet. 11 (4).
Within the cell, two replisomes proceed outward in opposite directions around the genome. Thus, bidirectional replication requires a replisome for each replicating fork. Fluorescent probes show that two replisomes are located near the middle of the growing cell (Fig. 3.28). The two copies of the DNA origin of replication (green in the figure), attached to the cell envelope, move apart as the cell expands. The termination site (red) remains in the middle of the cell, where the two replisomes continue replication at both forks. Finally, as the two replisomes approach each other, the termination site replicates. The two replisomes then separate from the DNA.
In a fast-growing cell, however, two new origin sites have already formed. At each new origin, two pairs of new replisomes have formed. The new origin sites begin a second round of replication, even before termination of the previous round. Slow-growing cells may instead have a pause before the next round of replication begins.
Completion of replication triggers Z-ring formation. For the cell to divide, DNA replication must be complete. During the process of replication, the cytoplasm contains several kinds of proteins that will determine septation, among them FtsZ (Fig. 3.28). Other septation-related proteins are bound to the cell membrane or to DNA. Replication of the DNA termination site triggers several proteins to form the divisome. For simplicity, only FtsZ is shown, as the subunits assemble to form the Z-ring.
Ultimately, septation completes cell division, and the two envelope ends come apart. In some species, such as filamentous cyanobacteria, the actual separation of cells may occur long after septation, forming extended filaments of individual cells. Others, such as Bacillus megaterium, commonly separate two or three generations after septation. Nutrient conditions may affect cell separation and cell size.
Thought Question
3.9 Suppose a cell has a defect in its ftsZ gene. What might happen to the cell during growth? How could such a mutant strain be maintained in the laboratory?
ANSWER
ANSWER
A cell with a defective ftsZ gene will fail to septate. As the cell grows, it expands and replicates its DNA, but no septum forms, and the daughter cells do not separate. Eventually, the cell’s nucleoids will entangle, and the long, filamented cell chain will die. There are several ways to maintain an ftsZ mutant in a viable state. One is to use a temperature-sensitive mutant, in which the FtsZ protein is functional at the permissive temperature but nonfunctional at the nonpermissive temperature. Another way is to maintain a copy of the ftsZ gene fused to a promoter that can be turned on or off by the presence of an inducer molecule such as a sugar (discussed in Chapter 10).
Septation of spherical cells. In spherical cells (cocci), such as Staphylococcus aureus, the process of septation generates most of the new cell envelope to enclose the expanding cytoplasm (Fig. 3.29A–C). The cell envelope pushes in, forming a ring that encircles the cell equator, as the completed envelope layers of the septum peel apart. Unlike rod-shaped cells (which elongate between divisions), the facing halves of each spherical cell form out of septum envelope as the two halves peel apart.
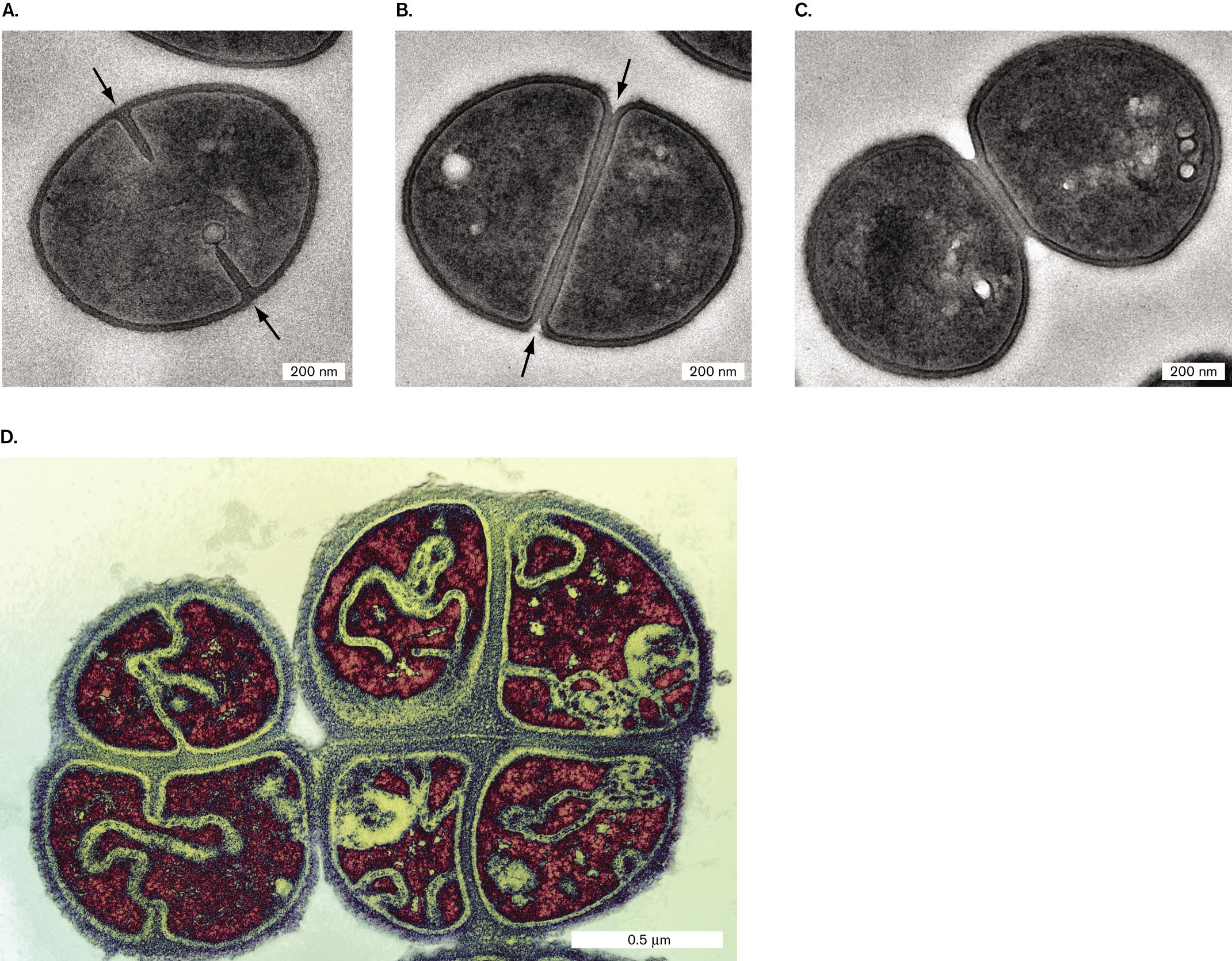
More information
Four transmission electron micrographs of septation without cell elongation.
A transmission electron micrograph of fission within Staphylococcus aureus. A coccus shaped cell of about 600 nanometers in diameter is visible. Two arrows identify the direction of fission towards the center of the cell. At opposing ends of the cell, the cell envelope is seen constricting towards the center.
A transmission electron micrograph of complete envelope partitioning in Staphylococcus aureus. A coccus shaped cell of about 600 nanometers in diameter is visible. The cell envelop completely cuts through the center of the cell to create two distinct sides of the cell. Arrows point from opposing sides of the cell to the center of the cell, describing the direction of division.
A transmission electron micrograph of Staphylococcus aureus daughter cells. Two cocci cells are visible in the final stages of separation. The cells have individual membranes and envelopes and are shown to be barely connected. Each cell has a diameter of about 500 nanometers.
A transmission electron micrograph of septation in Micrococcus tetragenus. Two M. tetragenus cells are visible, each about 1 micrometer in diameter. Septation is occurring along two planes in each cell, creating four distinct regions within each cell.
The spatial orientation of septation has a key role in determining the shape and arrangement of cocci. If the cell always septates in parallel planes, as in Streptococcus species, cells form chains. If, however, the cell septates in random orientations or if cells reassociate loosely after septation, they form compact hexagonal arrays similar to the grape clusters portrayed in classical paintings—hence the Greek-derived term staphylococci (staphyle means “bunch of grapes”). Such clusters are found in colonies of Staphylococcus aureus. If subsequent septation occurs at right angles to the previous division, the cells may form tetrads and even cubical octads called “sarcinae” (singular, sarcina). Tetrads are formed by Micrococcus tetragenus, a cause of pulmonary infections (Fig. 3.29D).
Bacterial cell size. How do cells “know” how large to grow? This question is hard to answer, as we are still discovering ever-smaller forms of life. An investigation of river bacteria, from Jillian Banfield’s lab at UC Berkeley, revealed tiny cells that pass through a 0.2-µm filter. These cells represent new kinds of life accounting for 15% of all taxa known at the time (discussed in Chapter 18). At the other end of the size range, the marine sulfur-oxidizing bacterium Thiomargarita namibiensis grows as a bubble of cytoplasm 200 µm across (presented in Chapter 1).
For a given species, cell size depends on genetic regulators and environmental constraints. When a bacterial population is first diluted into fresh medium, with abundant nutrients, cells elongate faster and reach larger sizes before septation and division. As nutrients become scarce, cell growth slows, and early division produces smaller cells. Thus, cell size is one factor in the phases of growth of bacterial populations (discussed in Chapter 4). Yet, repeated cycles of growth and starvation in minimal glucose medium lead E. coli populations to undergo selection for cells that are larger. Experimental evolution (discussed in Chapter 17) thus enables us to test models of cell size development.
To Summarize
- Bacterial cell division includes elongation and septation.
- DNA is organized in the nucleoid. In most bacterial species, the DNA is attached to the envelope at the origin of replication, on the cell’s equator. Loops of DNA called domains are supercoiled and bound to DNA-binding proteins.
- While DNA undergoes transcription, the growing RNA chain already binds ribosomes for translation. Newly transcribed RNA can be immediately translated, generating new proteins quickly.
- DNA is replicated bidirectionally by the replisome. During bacterial DNA replication, genes continue transcription and translation.
- Completion of DNA replication triggers Z-ring formation and septation. Septation may occur in one plane (forming a chain of cells) or at right angles to the previous septation (forming a tetrad).
- Bacterial cell size varies widely among taxa. Within a population, environmental parameters such as nutrient availability may determine cell size.
Glossary
- septation
- The formation of a septum, a new section of cell wall and envelope to separate two daughter cells.
- divisome
- A protein complex that manages the overall process of septation.
- domain
- 1. In taxonomy, one of three major subdivisions of life: Archaea, Bacteria, and Eukarya. 2. In protein structure, a portion of a protein that possesses a defined function, such as binding DNA. 3. In membranes, a region of membrane consisting of certain types of phospholipids that are distinct from surrounding lipids.
- DNA-binding protein
- A protein that binds to DNA and modulates its function.
- replisome
- A complex of DNA polymerase and other accessory molecules that performs DNA replication.
- staphylococcus
- A hexagonal arrangement of cells formed by septation in random orientations.
- Figure 3.13:
-
More information
A detailed illustration of peptidoglycan synthesis. Synthesis is shown to take place between the outer membrane and the inner membrane. Synthesis is mediated by a peptidoglycan synthesis complex, shown blown-up in the illustration. The complex extends from the interior of the cell across the inner membrane into the space between the inner and outer membranes. An arch shaped structure supports the complex, the structure is comprised of M r e B patches. Attached to the arch are several irregularly shaped proteins labeled M r e D, M r e C, R o d A, and R o d Z. Two penicillin binding proteins sit atop M r e C. The penicillin binding proteins are labeled P B P 2 and P B P 1 A. A chain of peptidoglycan is synthesized through the complex.
FIGURE 3.13 ■ Peptidoglycan synthesis is organized by penicillin-binding proteins (PBP2, PBP1A) and by cytoskeletal proteins. Protein MreB guides the direction of synthesis in helical arcs around the cell. - Fig. 3.12:
-
More information
A transmission electron micrograph of an isolated sacculus and an illustration of peptidoglycan structure.
A transmission electron micrograph of an isolated sacculus from Escherichia coli. The sacculus is a rectangular shape of about 20 micrometers in length and 10 micrometers in width. The sacculus appears transparent in the micrograph.
An illustration of peptidoglycan structure. It consists of several benzenes bonded vertically labeled as G and M alternatively. The text at the bottom reads L-Alanine, D-Glutamic acid, D-Alanine, and m-Diaminopimelic acid. G and M are shaded and is bonded with the alanine and acids. An arrow pointing toward the molecular structures of L-Alanine, D-Glutamic, D-Alanine acid, and m-Diaminopimelic acid. The structure of D-Alanine consists of C H in the center is single bonded to N H at the right, H 3 C at the left, and C O O H at the bottom. N H is single bonded to the structure of m-Diaminopimelic acid that consists of carbon in the center is single bonded to N H at the right, single bonded to carbon at the left, and single bonded to methyl at the bottom. The carbon in the left is double-bonded to oxygen at the top. Methyl at the bottom is single-bonded two methyl groups at the bottom and single bonded to C H C O O H is single bonded to N H. N H at the right is single bonded to the structure of D-Glutamic acid that consists of C H in the center is single bonded to N H at the right, carbon at the left, and (C H 2) 2 at the bottom and is single bonded to C O O H at the bottom. N H at the right is single bonded to the structure of L-Alanine that consists of C H in the center is single bonded to N H at the right, carbon at the left, and single bonded to methyl at the bottom. The carbon at the left is double-bonded to oxygen. Therefore the structures of L-Alanine, D-Glutamic, D-Alanine acid, and m-Diaminopimelic acid at the bottom are highlighted differently. N H at the bottom of the structure m-Diaminopimelic Acid is single bonded to carbon at the right in D-Alanine at the bottom. A bond between N H and Carbon reads Peptide cross-bridge forms with the release of D-alanine. They are blocked by penicillin. The text pointing toward the structure of D-Alanine reads Vancomycin binds D-Ala-D-Ala and blocks cross-bridge formation. An arrow from the D-Alanine structure leads to the structure that consists of C H in the center is single bonded to carbon in the right, single bonded to ammonia at the left, and double-bonded to oxygen at the right. C H in the center is single bonded to H 3 C at the top, and carbon at the right is single bonded to oxygen with minus ion at the top. Two structures of N-Acetylglucosamine and N-Acetylmuramic acid are bonded to the L-Alanine structure at the top right, and L-Alanine structure at the bottom left. The N-Acetylglucosamine structure consists of benzene in which C 1 is single bonded to C H 2 O H, C 2 is substituted by oxygen, C 3 is single bonded to hydrogen, C 4 is single bonded to N H at the bottom is single bonded to carbon at the bottom, in which carbon is single bonded to methyl at the right and double-bonded to oxygen at the bottom. C 5 is single bonded to hydrogen at the bottom, and C 6 is single bonded to hydroxyl and hydrogen. The N-acetylmuramic acid consists of benzene in which C 1 is single bonded to C H 2 O H, C 2 is substituted by oxygen, C 3 is single bonded to hydrogen, C 4 is single bonded to N H at the bottom is single bonded to carbon at the bottom, in which carbon is single bonded to methyl at the right and double-bonded to oxygen at the bottom. C 5 is single bonded to hydrogen at the bottom, and C 6 is single bonded to hydroxyl and hydrogen, in which hydrogens in C 6 of the two structures ate bonded to oxygen. C 5 of the N-Acetylmuramic acid is single bonded to oxygen at the bottom is single bonded to C H C H 3, which is single bonded to carbon and the carbon is double-bonded to oxygen at the bottom, and the carbon is single bonded to L- Alanine structure of N H at the right.
FIGURE 3.12 ■ The cell wall: peptidoglycan sugar chains and cross-bridges. A. Isolated sacculus (entire cell wall) from Escherichia coli (TEM). B. A disaccharide unit of glycan has an attached peptide of four to six amino acids. W. VOLLMER ET AL. 2008. FEMS MICROBIOL. REV. 32:149, FIG. 3A - Fig. 3.13:
-
More information
A detailed illustration of peptidoglycan synthesis. Synthesis is shown to take place between the outer membrane and the inner membrane. Synthesis is mediated by a peptidoglycan synthesis complex, shown blown-up in the illustration. The complex extends from the interior of the cell across the inner membrane into the space between the inner and outer membranes. An arch shaped structure supports the complex, the structure is comprised of M r e B patches. Attached to the arch are several irregularly shaped proteins labeled M r e D, M r e C, R o d A, and R o d Z. Two penicillin binding proteins sit atop M r e C. The penicillin binding proteins are labeled P B P 2 and P B P 1 A. A chain of peptidoglycan is synthesized through the complex.
FIGURE 3.13 ■ Peptidoglycan synthesis is organized by penicillin-binding proteins (PBP2, PBP1A) and by cytoskeletal proteins. Protein MreB guides the direction of synthesis in helical arcs around the cell. - Figure 3.23:
-
More information
Three illustrations and accompanying fluorescence micrographs describing shape determining proteins in different bacteria.
An illustration and an accompanying fluorescence micrograph of F t s Z in Staphylococcus. The cell is circular shaped, and inside the cell is a vertical oval ring labeled F t s Z. To the right is a microscopic image of F t s Z, or Z-ring, fluorescing in an E. coli bacterium. E. coli is a rod shaped cell of about 3 micrometers in length and 0.5 micrometer in width. F t s Z is identified as a bright band near the center of the cell.
An illustration and an accompanying fluorescence micrograph of F t s Z and M r e B in Escherichia coli. The cell is a long horizontal rod shape. The F t s Z ring is in the center, and overlapping and to the left and right are M r e B rings. To the right shows a microscopic fluorescent image of F t s Z bonded in the center of a cell and M r e B at the edges. The unit is about 2 micrometers long and 1 micrometer wide.
An illustration and an accompanying fluorescence micrograph of F t s Z, M r e B, and crescentin in Caulobacter crescentus. The cell is a long horizontal rod shape with a central bend. The bottom part of the cell where it curves is labeled Crescentin. F t s Z ring is located in the center of the cell and overlapping, and to the left and right are M r e B rings. To the right is a microscopic fluorescent image of a rod shaped cell with a central bend. The bend is fluorescent and labeled Crescentin. The cell is about 2 micrometers long and 1 micrometer wide.
FIGURE 3.23 ■ Shape-determining proteins. A. Cell diameter is maintained by FtsZ polymerization to form the Z-ring. B. Elongation of a rod-shaped cell requires MreB proteins. MreB polymerizes around an E. coli cell (MreB-YFP fluorescence) along with a Z-ring of FtsZ (fluorescent anti-FtsZ antibody). C. Crescent-shaped cells possess a third shape-determining protein, CreS (crescentin), which polymerizes along the inner curve of the crescent. Crescentin protein fused to green fluorescent protein (CreS-GFP) localizes to the inner curve of Caulobacter crescentus. Membrane-specific stain FM4-64 (red fluorescence) localizes to the membrane around the cell. Q. SUN AND W. MARGOLIN. 1998. J. BACTERIOL. 180:2050 PURVA VATS AND LAWRENCE ROTHFIELD. 2007. PNAS 104:17795 NORA AUSMEES ET AL. 2003. CELL 115:705