THE COMPARTMENTALIZATION OF CELLS
In this brief overview of the compartments of the cell and the relationships between them, we organize the cell’s organelles conceptually into a small number of discrete families, discuss how proteins are directed to specific organelles, and explain how proteins cross organelle membranes.
All Eukaryotic Cells Have the Same Basic Set of Membrane-enclosed Organelles
Many vital biochemical processes take place in membranes or on their surfaces. Membrane-bound enzymes, for example, catalyze lipid metabolism, and oxidative phosphorylation and photosynthesis both require a membrane to couple the transport of H+ to the synthesis of ATP. In addition to providing increased membrane area to host biochemical reactions, intracellular membrane systems form enclosed compartments that are separate from the cytosolic compartment, thus creating functionally specialized aqueous spaces within the cell. In these spaces, subsets of molecules (proteins, reactants, ions) are concentrated to optimize the biochemical reactions in which they participate. By having multiple types of compartments inside the same cell, biochemical reactions that require very different conditions, or would compete with each other, can nevertheless occur simultaneously. Because the lipid bilayer of cell membranes is impermeable to most hydrophilic molecules, the membrane of an organelle must contain membrane transport proteins to import and export specific metabolites. Each organellar membrane must also have a mechanism for importing, and incorporating into the organelle, the specific proteins that make the organelle unique.
Figure 12–1 illustrates the major intracellular compartments common to eukaryotic cells. The nucleus contains the genome (aside from mitochondrial and chloroplast DNA), and it is the principal site of DNA and RNA synthesis. The surrounding cytoplasm consists of the cytosol and the cytoplasmic organelles suspended in it. The cytosol constitutes a little more than half the total volume of the cell, and it is the main site of protein synthesis and degradation. It also performs most of the cell’s intermediary metabolism; that is, the many reactions that degrade some small molecules and synthesize others to provide the building blocks for macromolecules (discussed in Chapter 2).
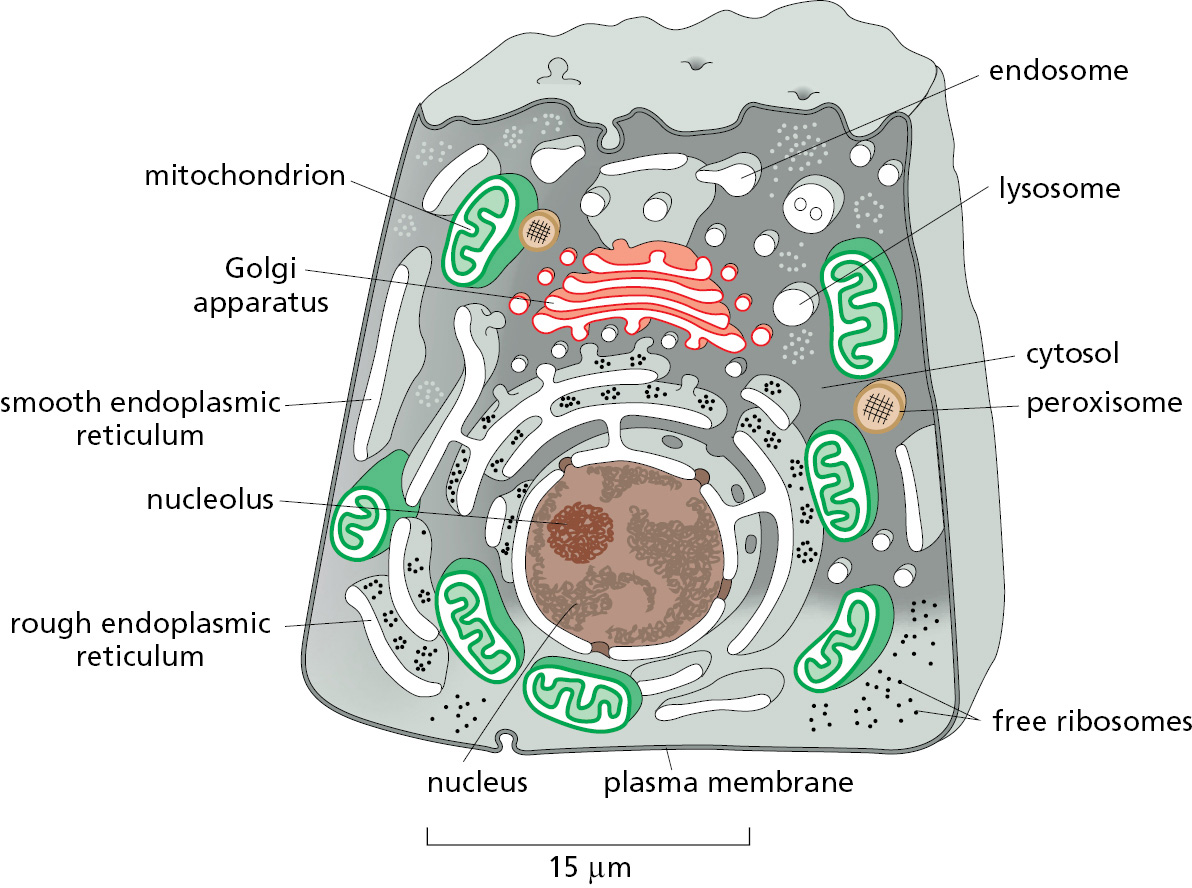
About half the total area of membrane in a eukaryotic cell encloses the labyrinthine spaces of the endoplasmic reticulum (ER). Most soluble and integral membrane proteins destined for the cell exterior or for other organelles are initially assembled at the ER. These proteins are transported into the ER as they are synthesized by ribosomes. The distinctive appearance in electron micrographs of ribosomes studding the surface of these regions of the ER is the reason they are termed the rough ER. The ER also produces most of the lipid and sterols for the rest of the cell and functions as a store for Ca2+ ions. These regions of the ER typically lack bound ribosomes and are called smooth ER.
The ER sends many of its proteins and lipids to the Golgi apparatus, which often consists of organized stacks of disc-like compartments called Golgi cisternae. The Golgi apparatus receives lipids and proteins from the ER and dispatches them to various destinations, usually covalently modifying them en route. Lysosomes contain digestive enzymes that degrade defunct intracellular organelles, as well as macromolecules and particles taken in from outside the cell by endocytosis. On the way to lysosomes, endocytosed material must first pass through a series of organelles called endosomes. As we will see, the ER, Golgi apparatus, lysosomes, endosomes, and plasma membrane are linked by the cell’s major pathways of membrane traffic.
Mitochondria and chloroplasts generate most of the ATP that cells use to drive reactions requiring an input of free energy; chloroplasts are a specialized version of plastids (present in plants, algae, and some protozoa), which can also have other functions, such as the storage of food or pigment molecules. Finally, peroxisomes are small vesicular compartments that contain enzymes used in various oxidative reactions.
On average, the membrane-enclosed compartments together occupy nearly half the volume of a cell (Table 12–1), and a large amount of intracellular membrane is required to make them. In liver and pancreatic cells, for example, the endoplasmic reticulum has a total membrane surface area that is, respectively, 25 times and 12 times that of the plasma membrane (Table 12–2). The membrane-enclosed organelles are packed tightly in the cytoplasm, and, in terms of area and mass, the plasma membrane is only a minor membrane in most eukaryotic cells (Figure 12–2).
TABLE 12–1 Relative Volumes Occupied by the Major Intracellular Compartments in a Liver Cell (Hepatocyte) |
|
Intracellular compartment |
Percentage of total cell volume |
Cytosol |
54 |
Mitochondria |
22 |
Rough ER cisternae |
9 |
Smooth ER cisternae |
5 |
Golgi cisternae |
1 |
Nucleus |
6 |
Peroxisomes |
1 |
Lysosomes |
1 |
Endosomes |
1 |
TABLE 12–2 Relative Amounts of Membrane Types in Two Kinds of Eukaryotic Cells |
||
Membrane type |
Percentage of total cell membrane |
|
Liver hepatocyte* |
Pancreatic exocrine cell* |
|
Plasma membrane |
2 |
5 |
Rough ER membrane |
35 |
60 |
Smooth ER membrane |
16 |
<1 |
Golgi apparatus membrane |
7 |
10 |
Mitochondria Outer membrane Inner membrane |
7 32 |
4 17 |
Nucleus Inner membrane** |
0.2 |
0.7 |
Secretory vesicle membrane |
Not determined |
3 |
Lysosome membrane |
0.4 |
Not determined |
Peroxisome membrane |
0.4 |
Not determined |
Endosome membrane |
0.4 |
Not determined |
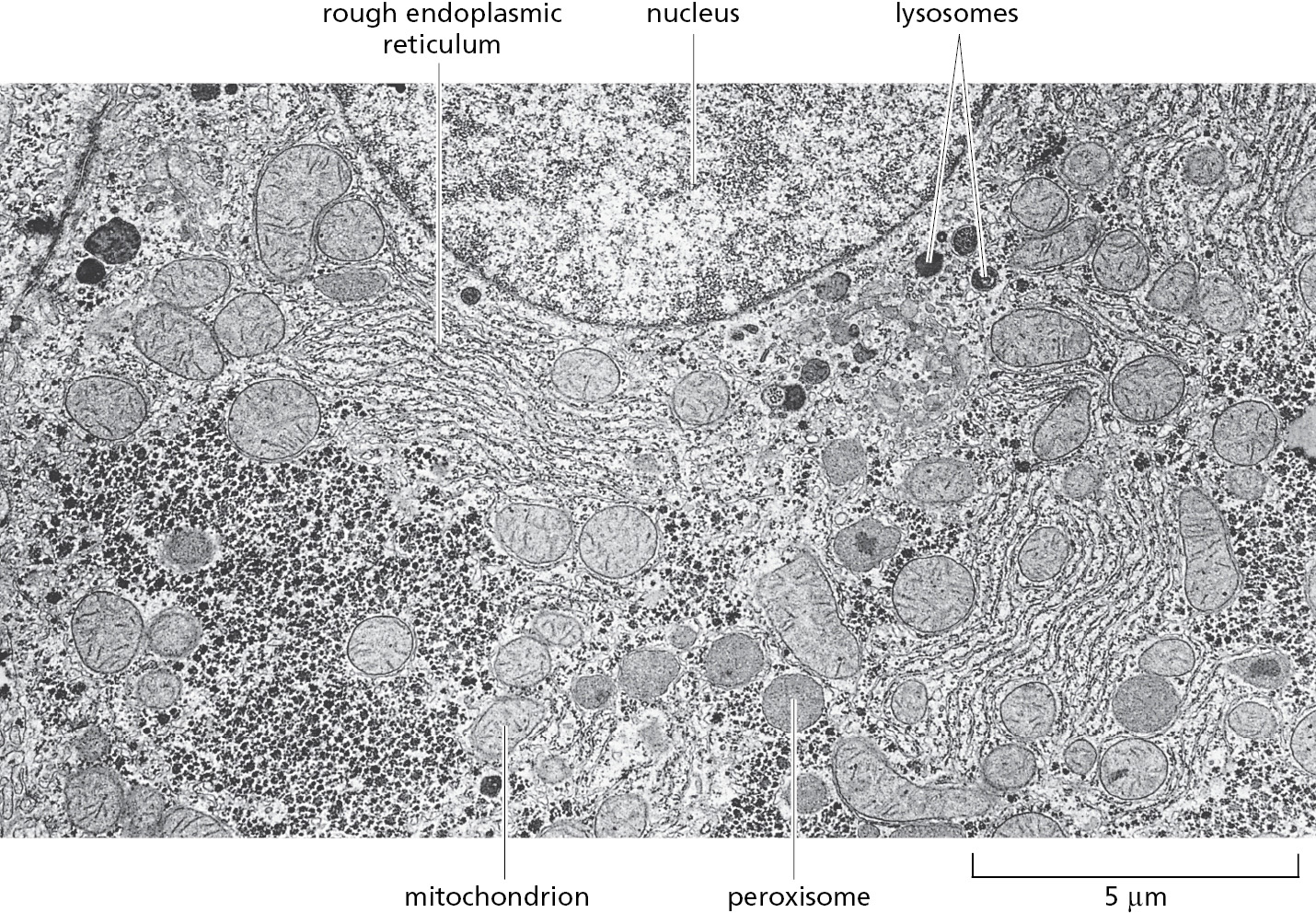
In general, each membrane-enclosed organelle performs the same set of basic functions in all cell types. But to serve the specialized functions of cells, these organelles vary in abundance and can have additional properties that differ from cell type to cell type. This is particularly apparent in cells that are highly specialized and therefore disproportionately rely on specific organelles. Plasma cells, for example, which daily secrete their own weight in antibody molecules into the bloodstream, contain vastly amplified amounts of rough ER, which is found in large, flat sheets. Cardiac muscle cells instead expand and specialize their smooth ER for Ca2+ storage and proliferate their mitochondria for energy production. Moreover, membrane-enclosed organelles are often found in characteristic positions in the cytoplasm. In most cells, for example, the Golgi apparatus is located close to the nucleus, whereas the network of ER tubules extends from the nucleus throughout the entire cytosol. These characteristic distributions depend on interactions of the organelles with the cytoskeleton (discussed in Chapter 16).
Evolutionary Origins Explain the Topological Relationships of Organelles
To understand the relationships between the compartments of the cell, it is helpful to consider how they might have originated. The precursors of the first eukaryotic cells are thought to have been relatively simple cells that—like most bacterial and archaeal cells—had a plasma membrane but no internal membranes. The plasma membrane in such cells provided all membrane-dependent functions, including the pumping of ions, ATP synthesis, protein secretion, and lipid synthesis. These ancestral precursors, like their modern-day prokaryotic counterparts,probably had a 1000- to 10,000-fold smaller volume than present-day eukaryotic cells. To increase in volume, the ancestral cells would have needed to maintain their surface area to volume ratio to sustain the many vital functions that membranes perform.
On the basis of the appearance of modern-day archaeal cells (see Figure 1–26), the membrane surface area might have initially increased by plasma membrane protrusions. The increased capacity to exchange metabolites with the surrounding environment via these protrusions would have facilitated symbiotic relationships with other organisms. Increased resource availability due to a combination of symbioses and membrane expansion may have allowed the evolution of progressively larger cells (Figure 12–3). Ultimately, the network of spaces between the numerous expanded protrusions would have become sealed off from the surrounding environment because of membrane fusion between protrusions. The consequences of this fusion are threefold and help to explain the major distinguishing features of eukaryotic cells.
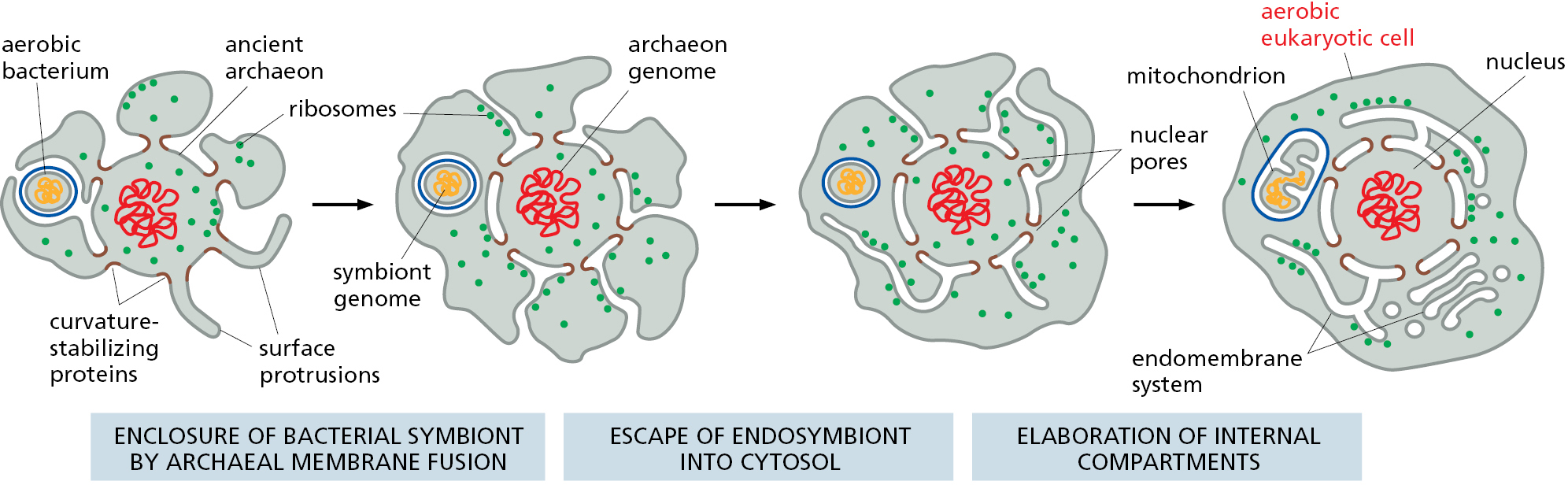
First, the cell now has a set of internal membranes that are derived from an ancestral prokaryotic plasma membrane. These internal membranes enclose interior spaces that are said to be topologically equivalent to each other and to the exterior of the cell (Figure 12–4), because they can communicate with one another, in the sense that molecules can get from one to the other without having to cross a membrane. We shall see that this topological relationship holds for all of the organelles involved in the secretory and endocytic pathways, including the ER, Golgi apparatus, endosomes, lysosomes, and peroxisomes. As we discuss in detail in the next chapter, their interiors communicate extensively with one another and with the outside of the cell via transport vesicles, which bud off from one organelle and fuse with another. In this way, proteins that enter the lumen of the ER can be secreted outside the cell.
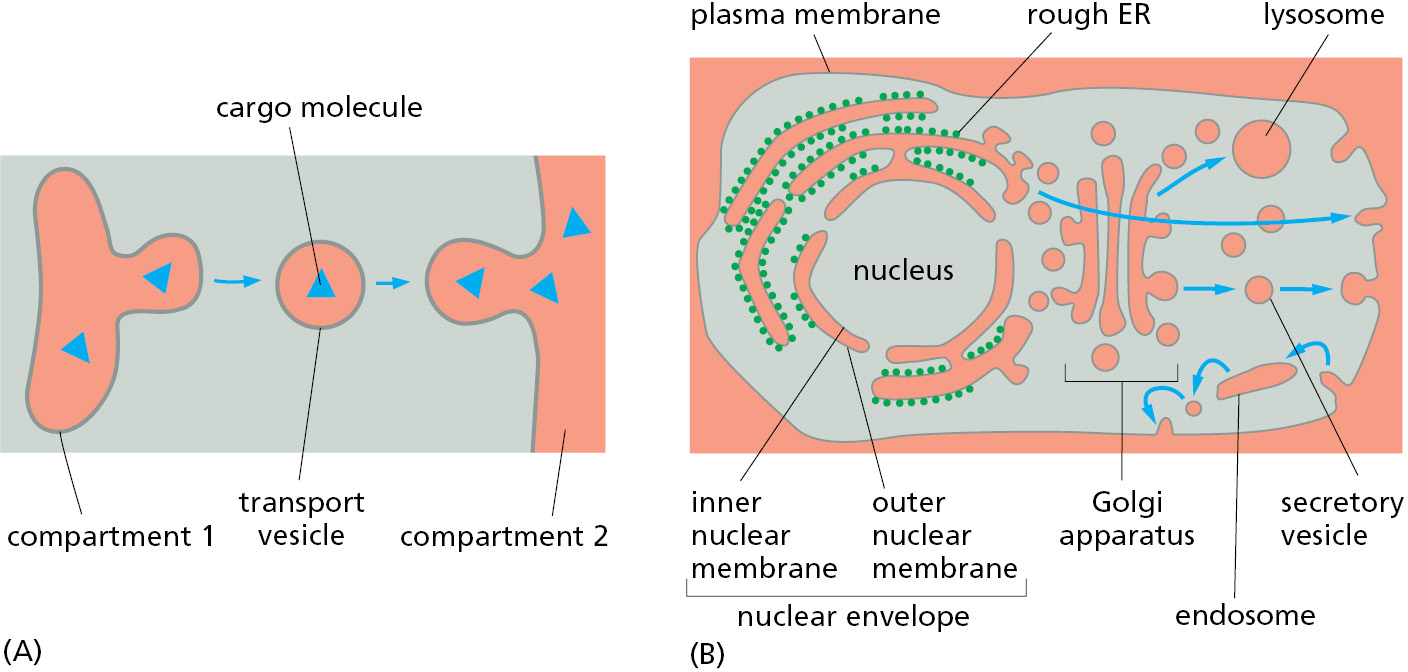
Second, the ancestral plasma membrane that surrounded the genome is now an internal membrane that becomes the inner nuclear membrane. Because of how it originated, the inner nuclear membrane is continuous with other plasma membrane–derived internal membranes, including the outer nuclear membrane. Specialized structures, the nuclear pore complexes, are located at points where the inner and outer nuclear membranes connect and provide a conduit for communication between the nucleus and cytosol. Segregation of an organism’s genetic material into a nucleus separate from the plasma membrane probably afforded greater protection from the environment. Furthermore, an expanded cytosol segregated from the nucleus would have facilitated the spatial separation of transcription from translation, thereby allowing greater regulation of gene expression by several mechanisms distinctive to eukaryotic cells.
Third, symbionts that were originally outside the cell were trapped inside the cell and became endosymbionts. At some point, endosymbionts escaped from their membrane enclosure into the cytosol where they eventually became mitochondria and plastids that contain their own genomes. The nature of these genomes and the close resemblance of the proteins in these organelles to those in some present-day bacteria provide strong evidence for their endosymbiont origins (see Figure 14–55). Like the bacteria from which they were derived, both mitochondria and plastids are enclosed by a double membrane, and they remain isolated from the extensive vesicular traffic that connects the interiors of most of the other membrane-enclosed organelles to each other and to the outside of the cell.
The evolutionary schemes we have outlined for the origins of eukaryotic organelles are most strongly supported by the striking similarities of the protein transport machinery of modern-day prokaryotes and eukaryotic organelles. The ability to transport proteins across and into membranes is a fundamental and essential feature of all living organisms. Thus, machinery that carries out these processes would have arisen in the earliest life-forms and been retained throughout evolution. The presence and orientation of these transport components therefore allow us to trace the origins and topology of the membranes within which they now reside. Consistent with the model for evolution of the endomembrane system of eukaryotic cells, the components that mediate protein import into the ER are homologous to the proteins that mediate export across the archaeal plasma membrane. Similarly, membrane protein insertion machinery in the outer and inner membranes of mitochondria and plastids contains homologous components found in the outer and inner membranes of various modern-day bacteria.
The major intracellular compartments in eukaryotic cells can therefore be categorized into three distinct families: (1) the nucleus and the cytosol, which are topologically equivalent (although functionally distinct) and connected by nuclear pore complexes; (2) all organelles that function in the secretory and endocytic pathways—including the ER, Golgi apparatus, endosomes, lysosomes, and the transport vesicles that move between them—and peroxisomes; (3) the endosymbiont-derived organelles: mitochondria and the plastids (in plants only).
Macromolecules Can Be Segregated Without a Surrounding Membrane
A membrane barrier is not the only way subsets of macromolecules can selectively segregate within cells. As we discussed in Chapter 3, one or more interacting proteins or nucleic acids can serve as scaffolds in biomolecular condensates (see Figure 3–77). These scaffold macromolecules create the condensate through multiple weak, fluctuating binding interactions among themselves; in addition, they recruit specific proteins and nucleic acids into the condensate as client macromolecules (Figure 12–5). Once recruited, the clients typically remain within the condensate because the local concentration of its binding sites on the interacting scaffold molecule is very high. Thus, when the client dissociates from a scaffold molecule, it is more likely to re-bind to another site on the scaffold molecule or to a neighboring one within the condensate than to diffuse away altogether. In this way, a specific set of proteins and nucleic acids can be concentrated into a cellular structure that excludes other surrounding macromolecules.
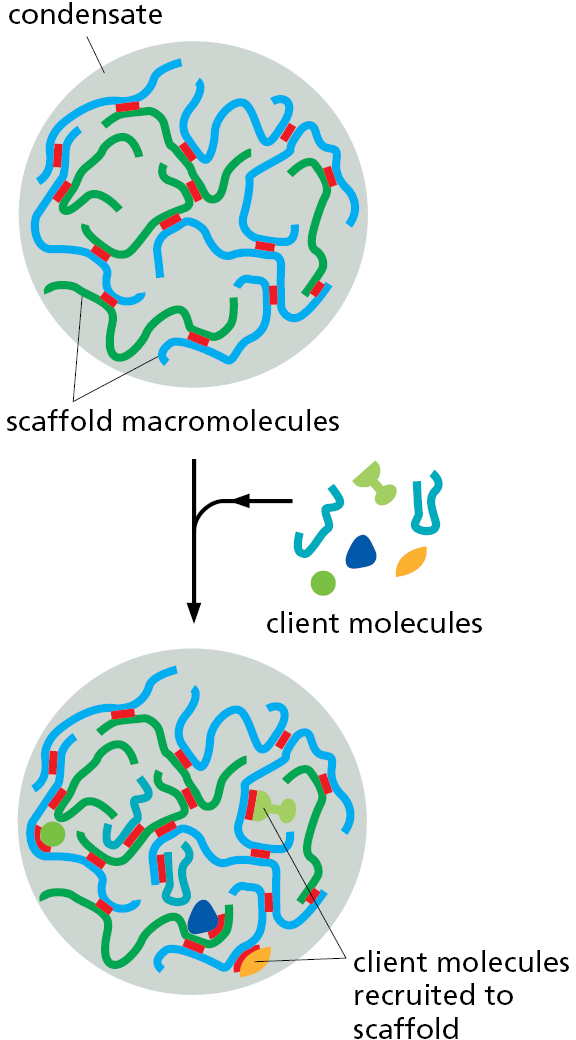
The largest and most conspicuous condensate in eukaryotic cells is the nucleolus, the structure within the nucleus where ribosomes are assembled (Movie 12.1). The central scaffolding component of the nucleolus is nascent pre-rRNA that is actively transcribed from arrays of rRNA genes. Nascent pre-rRNA recruits numerous proteins and small nucleolar RNAs (snoRNAs) required for pre-rRNA processing. These macromolecules further recruit other scaffold proteins—plus clients that include ribosomal proteins, assembly chaperones, and modification enzymes. In total, more than 400 proteins and RNAs contribute to the formation of this enormous condensate.
Biomolecular condensates can be found in all organisms (Figure 12–6), and eukaryotic cells contain a dozen or more different types (Table 12–3). The sizes of the known condensates range from ∼50 nm in diameter (slightly bigger than ribosomes) to a micrometer or more in the case of nucleoli. These structures include many different types of ribonucleoprotein condensates (some in the nucleus and others in the cytoplasm), a variety of different signaling protein clusters that can form tethered to the plasma membrane, and the “biochemical factories” that form as needed to catalyze DNA repair, DNA replication, and DNA transcription in the nucleus.
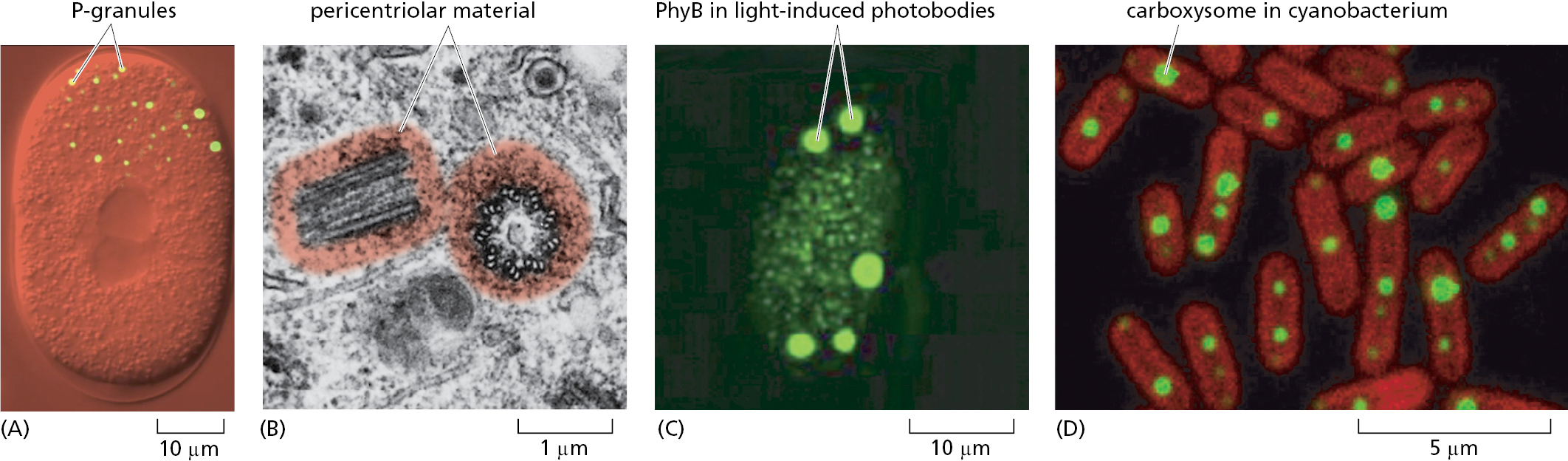
TABLE 12–3 Examples of Eukaryotic Biomolecular Condensates |
||
Biomolecular condensate |
Location |
Proposed associated function(s) |
Nucleolus |
Nucleus |
rRNA transcription and ribosome assembly |
Pyrenoid |
Chloroplast |
Carbon fixation from CO2 in algae |
Stress granules |
Cytosol |
Temporary storage, particularly of translation-related components |
P-granules |
Cytosol |
RNA metabolism and inheritance |
Balbiani body |
Cytosol |
Localization and inheritance of mRNAs and organelles |
Cajal body |
Nucleus |
mRNA processing |
Paraspeckles |
Nucleus |
Regulation of gene expression |
RNA transport granule |
Neuron |
RNA localization to subcellular locations in development and in neurons |
PML body |
Nucleus |
Storage of nuclear factors; regulation of gene expression |
Postsynaptic density |
Dendrite |
Organization of macromolecules needed for neuronal transmission |
As illustrated by the nucleolus, each type of condensate is enriched for a characteristic complement of proteins (and in many cases, nucleic acids) that interact with each other to maintain the condensate’s identity and integrity. The specificity of at least a subset of macromolecular interactions within the condensate ensures that it remains distinct in its composition and function. Thus, biomolecular condensates and membrane-enclosed compartments represent two different mechanisms that are used by eukaryotic cells to segregate subsets of macromolecules that execute specialized biochemistry (see Table 3–3). Because of this conceptual similarity, condensates are sometimes referred to as membraneless organelles. Historically, organelles were intracellular structures that could be directly visualized in the light or electron microscope. This is why the nucleolus and centrosome are called organelles. Most condensates are not organelles by this historical definition; nevertheless, they are cellular structures that segregate and concentrate specific macromolecules.
Multivalent Interactions Mediate Formation of Biomolecular Condensates
The formation of a biomolecular condensate requires that at least one of its constituent macromolecules engage in a set of weak, multivalent interactions with either itself or other constituents (see Figure 3–77A). The sites of these interactions are often separated by flexible and unstructured regions of the macromolecule. For example, nascent pre-rRNA in the nucleolus is a flexible molecule that binds a variety of clients and other scaffold molecules at numerous points along its length. Similarly, the scaffolding proteins that generate condensates of signaling proteins under the plasma membrane typically contain multiple protein–protein interaction domains separated by flexible intrinsically disordered regions that lack secondary structure. Experiments with artificial multivalent proteins have shown that this is the minimal element needed to drive condensate formation in a test tube and in cells.
Each individual interaction within a condensate is often very weak, allowing the macromolecules to rapidly exchange their relative positions. These dynamic and frequent rearrangements, together with the structural flexibility of many condensate constituents, means that the molecules within the condensate are highly mobile and do not have fixed positions relative to each other. This property causes the condensate to behave as a liquid. As discussed in Chapter 3 (pp. 171–173), despite this liquidlike property, the condensate does not dissolve into its surroundings because the interaction energy within the condensate offsets the entropy that would be gained if the molecules were dispersed. This is how the condensate can remain a liquid that stably resides within another liquid (the cytosol), a phenomenon termed “liquid–liquid phase separation.”
Different noncovalent chemical bonds can form the weak interactions between macromolecules that drive condensate formation. Cation–pi interactions, pi–pi interactions, charge–charge interactions, short regions of crossed β sheets, and short stretches of nucleic acid base-pairing can all contribute to condensate formation. The key requirement is that the interactions have sufficient binding energy to offset the loss of entropy caused by association, while being sufficiently dynamic to give the condensate a liquid character. When these requirements are met, condensates have a spherical shape, deform and flow under shear force, and can undergo fusion and fission.
If the interactions in a condensate become less dynamic as incrementally more stable interactions form over time, its properties can change to that of a gel and eventually a solid where the binding interactions remain fixed. There is a continuum across the spectrum of physical properties that characterize different condensates. Cells can exploit such differences by forming a condensate within a condensate. This can occur if a subset of macromolecules within a condensate has slightly higher affinity among its macromolecules than for other macromolecules of the condensate. This subset then forms a new condensate with distinct physical properties (Figure 12–7). This is how the nucleolus is thought to be segregated into morphologically different concentric shells, each of which is enriched for subsets of nucleolar proteins dedicated to different aspects of ribosome assembly.
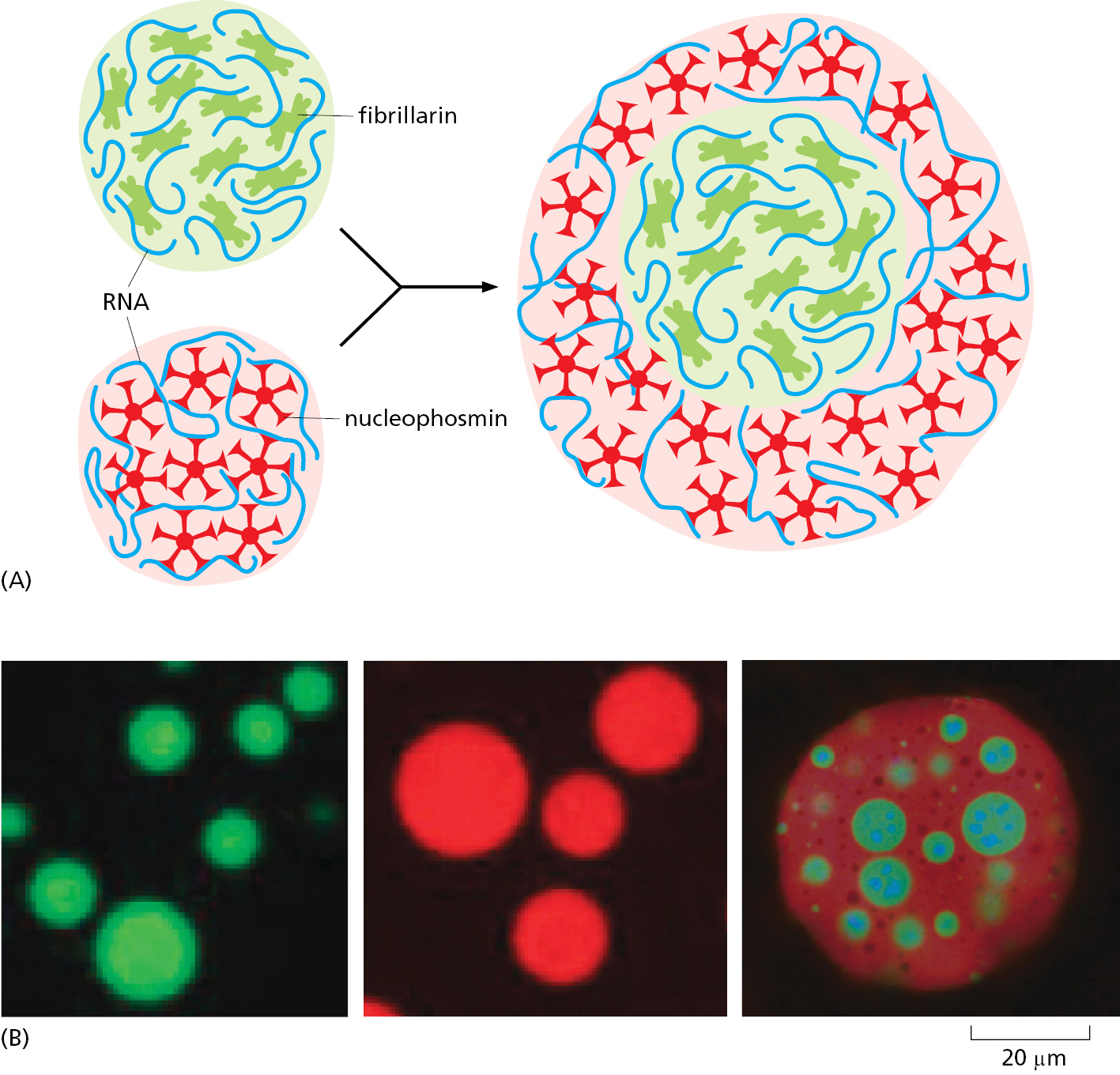
Biomolecular Condensates Create Biochemical Factories
The fluctuating network of interactions among the macromolecules inside of a condensate excludes other macromolecules from the surrounding environment. By contrast, nucleotides, metabolites, cofactors, and other small molecules can rapidly diffuse into the condensate where they can engage with the enzymes that reside there. The product of these enzymes within the condensate can be used by other enzymes that are coresident in the condensate before the product diffuses away. In this way, multistep reactions can be accelerated to rates beyond that possible without co-segregation of the enzymes inside a condensate.
Consider for example, the pyrenoid, a complex structure found in many algae that contains a condensate enriched in the enzyme ribulose 1,5-bisphosphate carboxylase/oxygenase (Rubisco) and a pyrenoid-specific scaffolding protein. The Rubisco-containing condensate is interwoven with membrane tubules. Carbonic anhydrase inside the membrane tubule converts bicarbonate (HCO3–) into CO2, which Rubisco uses to carboxylate ribulose 1,5-bisphosphate (Figure 12–8). This carboxylation reaction is a critical early step in carbon fixation during photosynthesis (discussed in Chapter 14). If Rubisco were not in a condensate in close proximity to carbonic anhydrase, the low free CO2 concentration combined with a competition by oxygen for Rubisco’s active site would favor reaction with oxygen (termed “photorespiration”; see Chapter 14, p. 847) over carbon fixation. Land plants do not need a pyrenoid to fix carbon because CO2 in the air is more plentiful than the very low concentration of dissolved CO2 available as HCO3– to algae living in aquatic environments. The pyrenoid illustrates how the segregation of sequential biochemical reactions into a condensate can both speed reactions and minimize alternate off-pathway outcomes. In the same way, carrying out the highly complex and ordered process of ribosome assembly within the nucleolus prevents unwanted side reactions while promoting the desired ones.
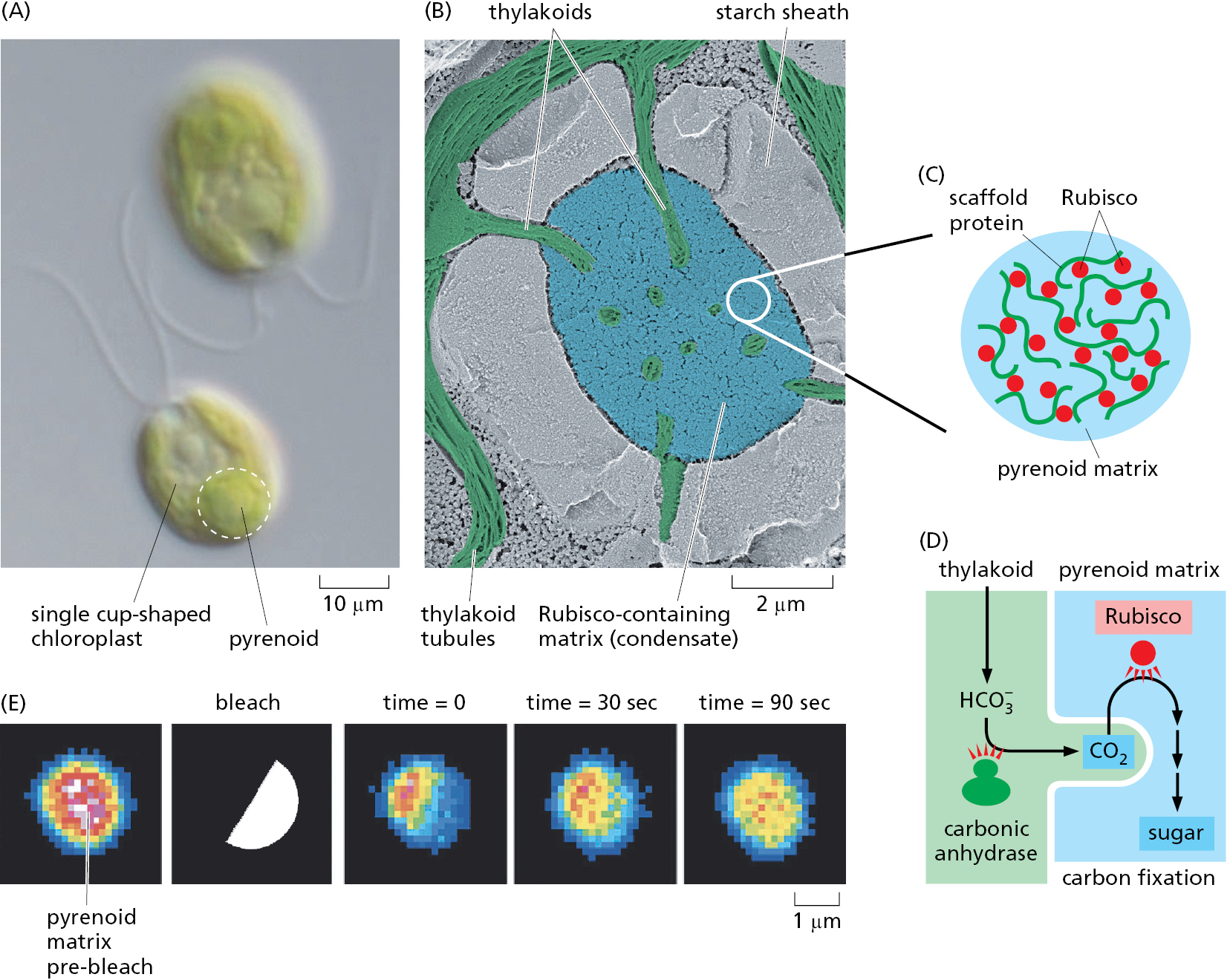
Scientists can produce artificial condensates that contain a desired set of macromolecules by engineering them with multivalent interaction domains. This approach can be used to experimentally enhance the efficiency of an otherwise unfavorable reaction. In one experiment, all of the factors required to misread a UAG stop codon as a sense codon were engineered with artificial multivalent interaction modules to generate a condensate inside the cell. The UAG codon of the mRNA within this condensate was efficiently interpreted as a sense codon, while other mRNAs in the surrounding cytosol terminated at UAG codons. This experiment illustrates the minimal features needed to produce a condensate-based biochemical factory within a cell and that such features can be rationally designed and engineered.
Biomolecular Condensates Form and Disassemble in Response to Need
As we have seen, the formation and stability of a biomolecular condensate rely on weak interactions among its constituents overcoming the entropy of a well-mixed system. This means that even small changes in the strength of interactions can influence the formation and physical properties of the condensate. The formation and dissolution of a condensate can therefore be readily controlled by changing the strength of the multivalent interactions that mediate its assembly. This is often accomplished by post-translational modifications, such as phosphorylation, and this mechanism is commonly used to rapidly form and disassemble large signaling clusters at the plasma membrane (Figure 12–9). Condensate formation and disassembly can also be induced by a change in a cellular condition such as temperature, pH, or osmolarity. The reversibility of condensate formation is used by cells to regulate condensates in response to need, and it affords the cell flexibility and speed in adapting to changing needs.
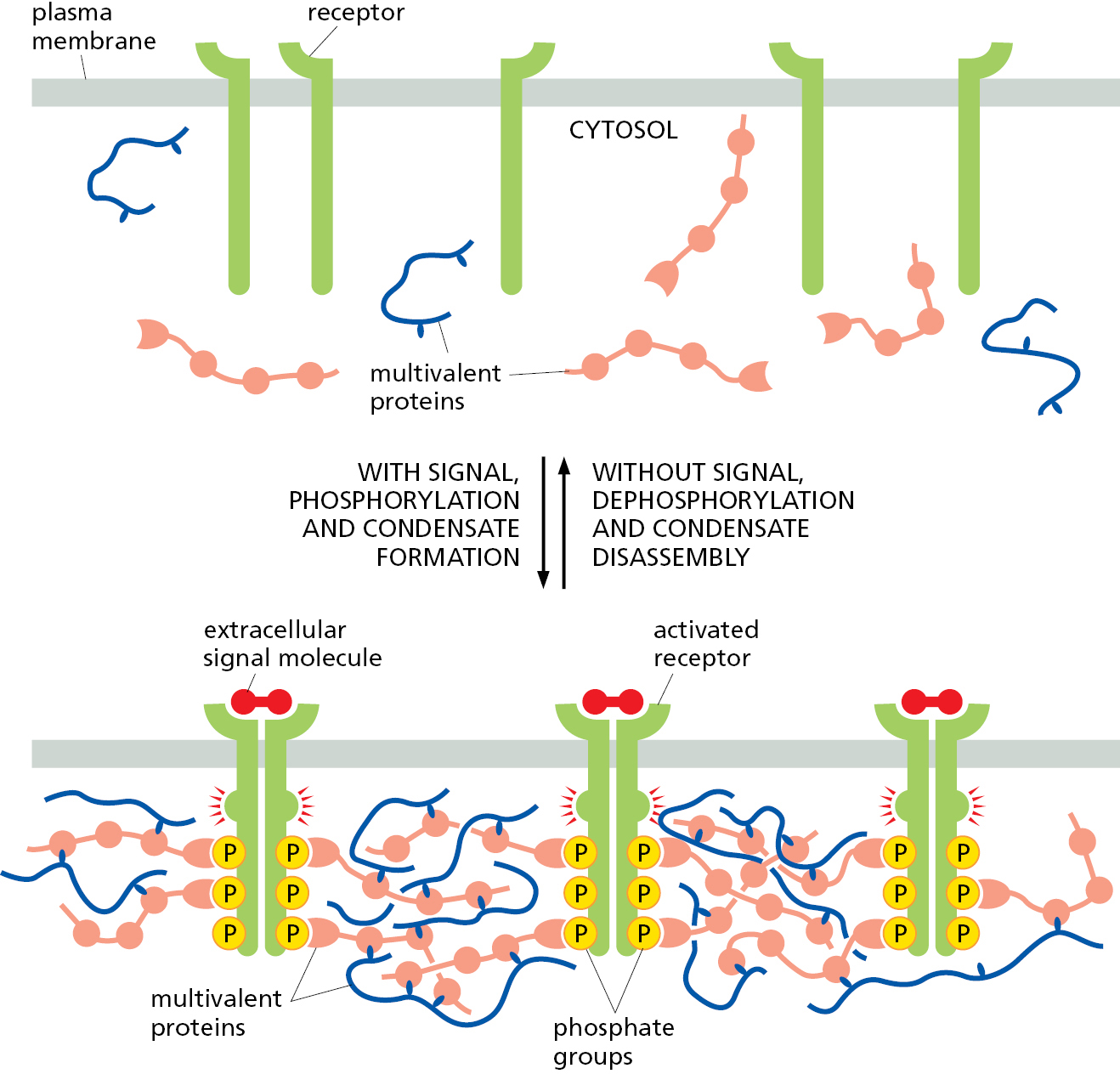
For example, the condensates called stress granules only form during certain types of cellular stress, and they dissolve when the stress is alleviated. These condensates are enriched in translationally inactive mRNAs, various translation factors, ribosomal subunits, and various RNA-binding proteins. They form when a block in translation initiation exposes mRNA regions that would normally be covered by translating ribosomes. When these mRNAs become exposed, they can interact with each other and with RNA-binding proteins to nucleate a condensate. It is thought that the resulting condensates serve as a storage depot for these mRNAs and factors when they are not being actively used. By temporarily sequestering these macromolecules during stress rather than degrading them, the cell can avoid the need to produce them de novo once the stress has been resolved.
Proteins Can Move Between Compartments in Different Ways
Nearly all proteins, except a few inside mitochondria and plastids, begin their synthesis on ribosomes in the cytosol. The final location of each protein depends on its amino acid sequence, which can contain one or more sorting signals that direct its delivery to different parts of the cell. The sorting signals in the transported protein are recognized by complementary sorting receptors that mediate movement between compartments. By contrast, proteins that do not have any sorting signals remain in the cytosol as permanent residents. There are four fundamentally different ways a protein is moved from one compartment to another. These four mechanisms are described below, and the transport steps at which they operate are outlined in Figure 12–10. We discuss protein translocation and gated transport in this chapter, vesicular transport in Chapter 13, and engulfment in both this chapter and the next.
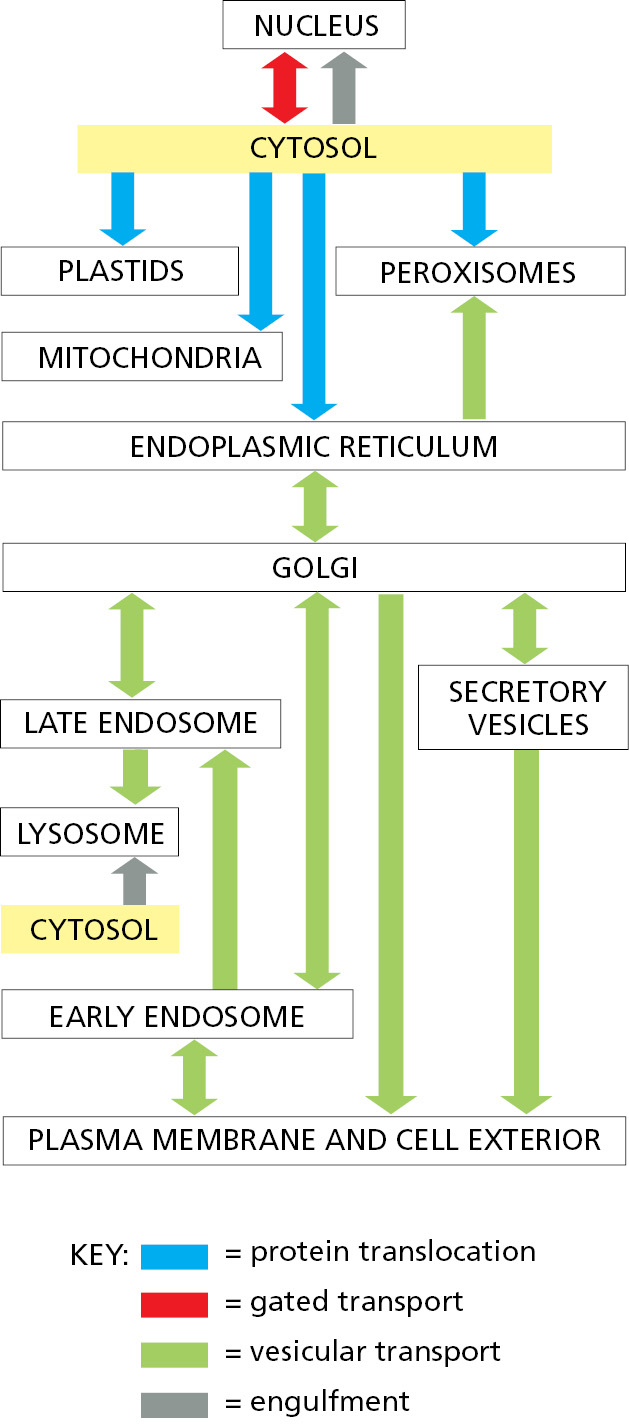
- In protein translocation, transmembrane protein translocators directly transport specific proteins from the cytosol into a space that is topologically distinct: either the other side of a membrane or within the lipid bilayer in the case of integral membrane proteins. The transported protein molecule usually must unfold to snake through the translocator. The initial transport of selected proteins from the cytosol into the ER lumen, the ER membrane, or mitochondria occurs in this way.
- In gated transport, proteins and RNA molecules move between the cytosol and the nucleus through nuclear pore complexes in the nuclear envelope. The nuclear pore complexes function as selective gates that support the active transport of specific macromolecules and macromolecular assemblies between the two topologically equivalent spaces.
- In vesicular transport, membrane-enclosed transport intermediates—which may be small, spherical transport vesicles, elongated tubules, or larger, irregularly shaped fragments of organelles—ferry proteins from one topologically equivalent compartment to another. The transport intermediate becomes loaded with a cargo of molecules derived from the lumen and membrane of the originating compartment as it buds and pinches off. At the destination compartment, the transport intermediate fuses with the compartment’s enclosing membrane to discharge its cargo (Figure 12–11). The transfer of soluble and membrane-embedded proteins from the ER to the Golgi apparatus, for example, occurs in this way. The proteins transported by vesicular transport never cross a membrane during the process, and therefore retain their topological relationships within the cell.
Figure 12–11 Vesicle budding and fusion during vesicular transport. Transport vesicles bud from one compartment (donor) and fuse with another topologically equivalent (target) compartment. In the process, a subset of soluble components (red dots) are transferred from lumen to lumen. Note that membrane is also transferred and that the original orientation of both proteins and lipids in the donor compartment membrane is preserved in the target compartment membrane. Thus, membrane proteins retain their asymmetrical orientation, with the same domains always facing the cytosol. - In engulfment, such as autophagy (discussed in Chapter 13), double-membrane sheets wrap around portions of the cytoplasm often including fragments of organelles or even entire organelles (Figure 12–12). This membrane structure then seals by membrane fusion to enclose a separate compartment, the autophagosome. The re-formation of the nuclear envelope after mitosis (discussed later in this chapter) follows a conceptually similar process. ER tubes and sheets wrap around decondensing chromosomes and then fuse laterally with one another to form a sealed double-membrane envelope only traversed by the nuclear pores.
Figure 12–12 Formation of a new compartment by engulfment of contents inside of a membrane.
In addition to these mechanisms for protein movement into and between membrane-enclosed compartments, a simpler mechanism based on direct physical binding is used by macromolecules to enter biomolecular condensates. In this mechanism, the macromolecule specifically binds to another protein or RNA that is already part of the condensate to which it is specifically recruited. Once recruited, the macromolecule remains within the condensate because of persistent and repeated interactions with its partner. The interaction between a macromolecule and its binding partner in the condensate is analogous to the interaction between a sorting signal and its cognate sorting receptor; in both cases, the interaction specifies the macromolecule’s destination.
Sorting Signals and Sorting Receptors Direct Proteins to the Correct Cell Address
Sorting signals are usually composed of amino acid side chains in a protein and come in two general varieties: a linear sequence of amino acids (called a signal sequence) or a specific three-dimensional arrangement of amino acids (called a signal patch). Sorting signals for protein translocation into organelles are linear signal sequences, while examples of linear signals and signal patches are known for nuclear and vesicular transport. The linear signal sequences for protein translocation are often found at the N-terminus of the polypeptide chain. These N-terminal signal sequences are usually removed from the finished protein by specialized signal peptidases once the sorting process is complete. Other types of signal sequences are not removed and remain part of the final mature protein.
Each signal sequence specifies a particular destination in the cell. The signal sequence for initial transfer to the ER usually includes a linear sequence of about 5–10 predominantly hydrophobic amino acids. Many of these proteins will in turn pass from the ER to the Golgi apparatus, but those with a specific signal sequence of four amino acids at their C-terminus are recognized as ER residents and are returned to the ER. Proteins destined for mitochondria have signal sequences of yet another type, in which positively charged amino acids alternate with hydrophobic ones. The signal for protein import into the nucleus is composed primarily of positively charged amino acids. Finally, many proteins destined for peroxisomes have a signal sequence of three characteristic amino acids at their C-terminus. A sorting signal for any particular destination needs to be sufficiently distinctive from all other sequences to permit its selective recognition by the appropriate sorting receptor.
Figure 12–13 presents some specific signal sequences. Experiments in which the peptide is transferred from one protein to another by genetic engineering techniques have demonstrated the importance of each of these signal sequences for protein targeting. Placing the N-terminal ER signal sequence at the beginning of a cytosolic protein, for example, redirects the protein to the ER; removing or mutating the signal sequence of an ER protein causes its retention in the cytosol. Signal sequences are therefore both necessary and sufficient for protein targeting. Even though their amino acid sequences can vary greatly, the signal sequences of proteins having the same destination are often functionally interchangeable; in these instances, physical properties, such as hydrophobicity, are more important in the signal-recognition process than the exact amino acid sequence.
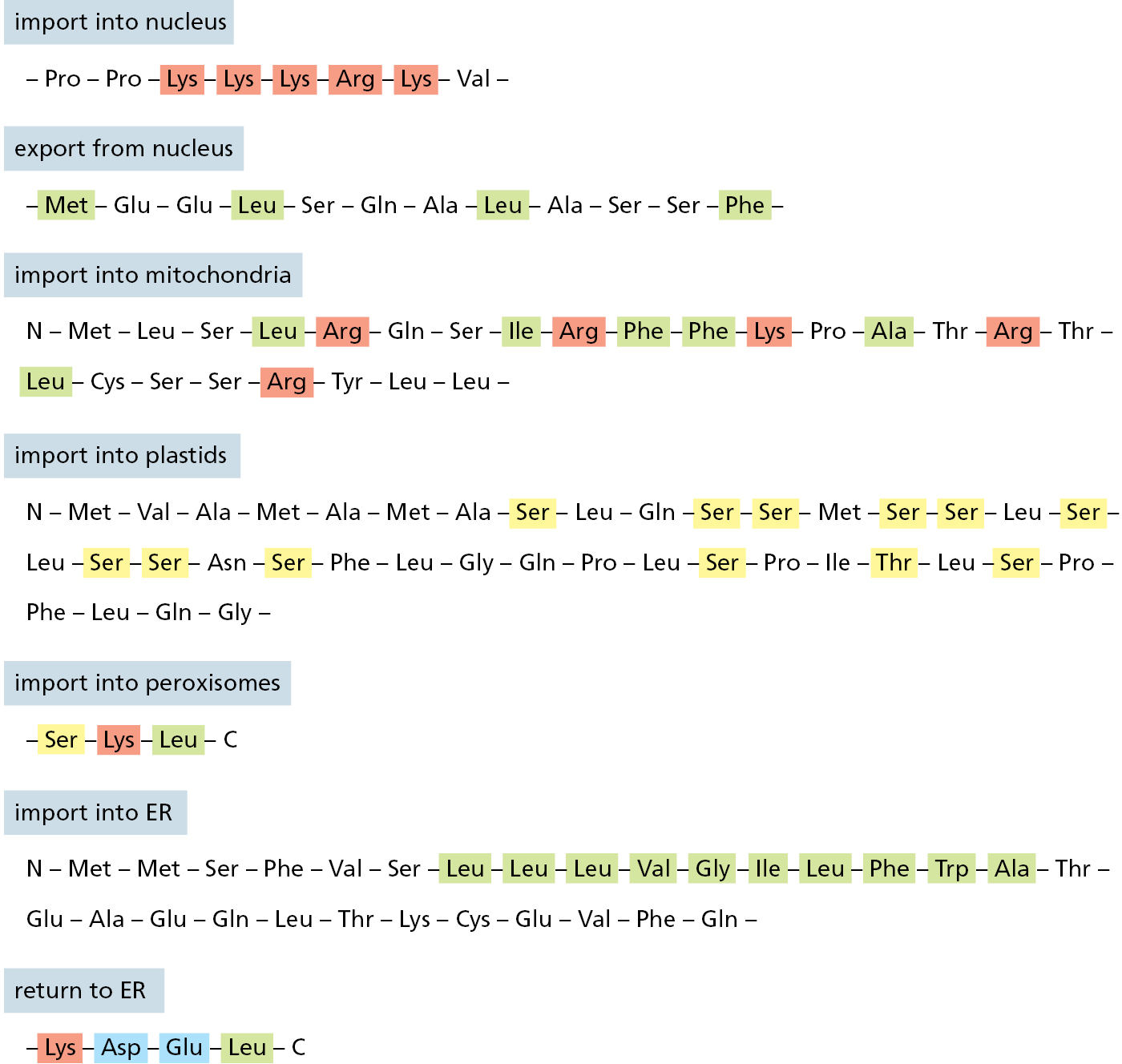
Sorting signals are recognized by complementary sorting receptors that guide proteins to their appropriate destination, where the receptors unload their cargo. The receptors function catalytically: after completing one round of targeting, they return to their point of origin to be reused. Most sorting receptors recognize classes of proteins rather than an individual protein species. They can therefore be viewed as public transportation systems, dedicated to delivering numerous different components to their correct locations in the cell.
Construction of Most Organelles Requires Information in the Organelle Itself
When a cell reproduces by division, it has to duplicate its chromosomes, its enclosing plasma membrane, and its organelles. In general, cells do this by expanding the plasma membrane and organelles with new proteins and lipids before division and segregation to the two daughter cells. The delivery of new proteins for growth of the ER, mitochondria, and plastids requires preexisting organelle-specific protein translocators. Because the incorporation of new protein translocators requires a preexisting protein translocator, a cell must already have at least some functional ER to make more ER; the same applies to mitochondria and plastids. Thus, two types of information are required to construct these organelles: the DNA that specifies an organelle’s proteins and preexisting protein translocator(s) in the organellar membrane for incorporating new deliveries of protein. Both types of information are passed from parent cell to daughter cells to maintain the cell’s compartmental organization.
Some organelles, such as lysosomes, acquire all of their proteins and membrane by vesicular transport from other organelles (see Chapter 13). Because it is possible, in principle, to construct such organelles de novo, they do not necessarily have to be inherited at cell division. Similarly, biomolecular condensates can be constructed de novo by self-assembly of the constituent proteins and nucleic acids. Thus, during cell division, a condensate can be disassembled, its constituents distributed stochastically among the two daughter cells, then reassembled into a condensate. This is how the nucleolus is acquired by daughter cells.
Summary
Eukaryotic cells contain intracellular membrane-enclosed organelles that make up nearly half the cell’s total volume. The main ones present in all eukaryotic cells are the endoplasmic reticulum, Golgi apparatus, nucleus, mitochondria, lysosomes, endosomes, and peroxisomes; plant cells also contain plastids such as chloroplasts. All organelles contain distinct sets of proteins, which mediate each organelle’s unique function.
Cells can also segregate subsets of their macromolecules into biomolecular condensates such as the nucleolus. The components inside these condensates can work together to carry out specialized biochemical reactions. The cell contains a dozen or more condensates that vary widely in size and can assemble and disassemble in response to need.
Each newly synthesized organellar protein must find its way from a ribosome in the cytosol, where the protein is made, to the organelle where it functions. It does so by using sorting signals in its amino acid sequence that are recognized by complementary sorting receptors, which deliver the protein to the appropriate target organelle. Proteins that function in the cytosol do not contain sorting signals and therefore remain there after they are synthesized.
During cell division, organelles such as the ER and mitochondria are distributed to each daughter cell. These organelles contain information that is required for their construction, and so they cannot be made de novo. Biomolecular condensates can be constructed de novo because they self-assemble from components that are encoded genetically.
Glossary
- cytoplasm
- Contents of a cell that are contained within its plasma membrane but, in the case of eukaryotic cells, outside the nucleus.
- lumen
- The space inside a hollow structure. In cells: the cavity enclosed by an organelle membrane. In tissues: the cavity enclosed by a sheet of cells.
- membraneless organelles
- An assembly of specific proteins held together by multivalent, low-affinity interactions. Also referred to as a biomolecular condensate.
- sorting signals
- Signal sequence or signal patch that directs the delivery of a protein to a specific location, such as to a particular intracellular compartment.
- protein translocation
- The process of moving a protein across a membrane.
- gated transport
- Movement of proteins between the cytosol and the nucleus through the nuclear pore complexes in the nuclear envelope; these complexes function as selective gates.
- vesicular transport
- The transport of proteins from one cell compartment to another by means of membrane-bounded intermediaries, such as vesicles or organelle fragments.
- engulfment
- Process by which a portion of the cytoplasm becomes enclosed by a double membrane, such as during autophagy.
- signal sequences
- Short continuous sequence of amino acids that determines the eventual location of a protein in the cell. An example is the N-terminal sequence of 20 or so amino acids that directs nascent secretory and transmembrane proteins to the endoplasmic reticulum.
- signal patches
- Protein-sorting signal that consists of a specific three-dimensional arrangement of amino acids on the folded protein’s surface.
- signal peptidases
- Enzyme that removes a terminal signal sequence from a protein once the sorting process is complete. (Figures 12–27 and 12–49)
- sorting receptors
- Proteins involved in binding signal sequences or cargo proteins and bringing them to specific sites in the cell where cargo proteins are released. Sorting receptors pick up and deliver cargo proteins repeatedly.
Endnotes
- These two cells are of very different sizes: the average hepatocyte has a volume of about 5000 μm3 compared with 1000 μm3 for the pancreatic exocrine cell. Total cell membrane areas are estimated at about 110,000 μm2 and 13,000 μm2, respectively. Return to reference *
- These two cells are of very different sizes: the average hepatocyte has a volume of about 5000 μm3 compared with 1000 μm3 for the pancreatic exocrine cell. Total cell membrane areas are estimated at about 110,000 μm2 and 13,000 μm2, respectively. Return to reference *
- The outer nuclear membrane is included in the measurement of the rough ER and is roughly equal to the inner membrane. Return to reference **