THE ENDOPLASMIC RETICULUM
The membrane of the endoplasmic reticulum (ER) typically constitutes more than half of the total membrane of an average animal cell (see Table 12–2). The ER is organized into a netlike labyrinth of branching tubules and flattened sacs that extends throughout the cytosol (Figure 12–14 and Movie 12.2). The tubules and sacs interconnect, and their membrane is continuous with the outer nuclear membrane. This membrane system encloses a single internal space, called the ER lumen, which is continuous with the space between the inner and outer nuclear membranes. The ER often occupies more than 10% of the total cell volume (see Table 12–1).
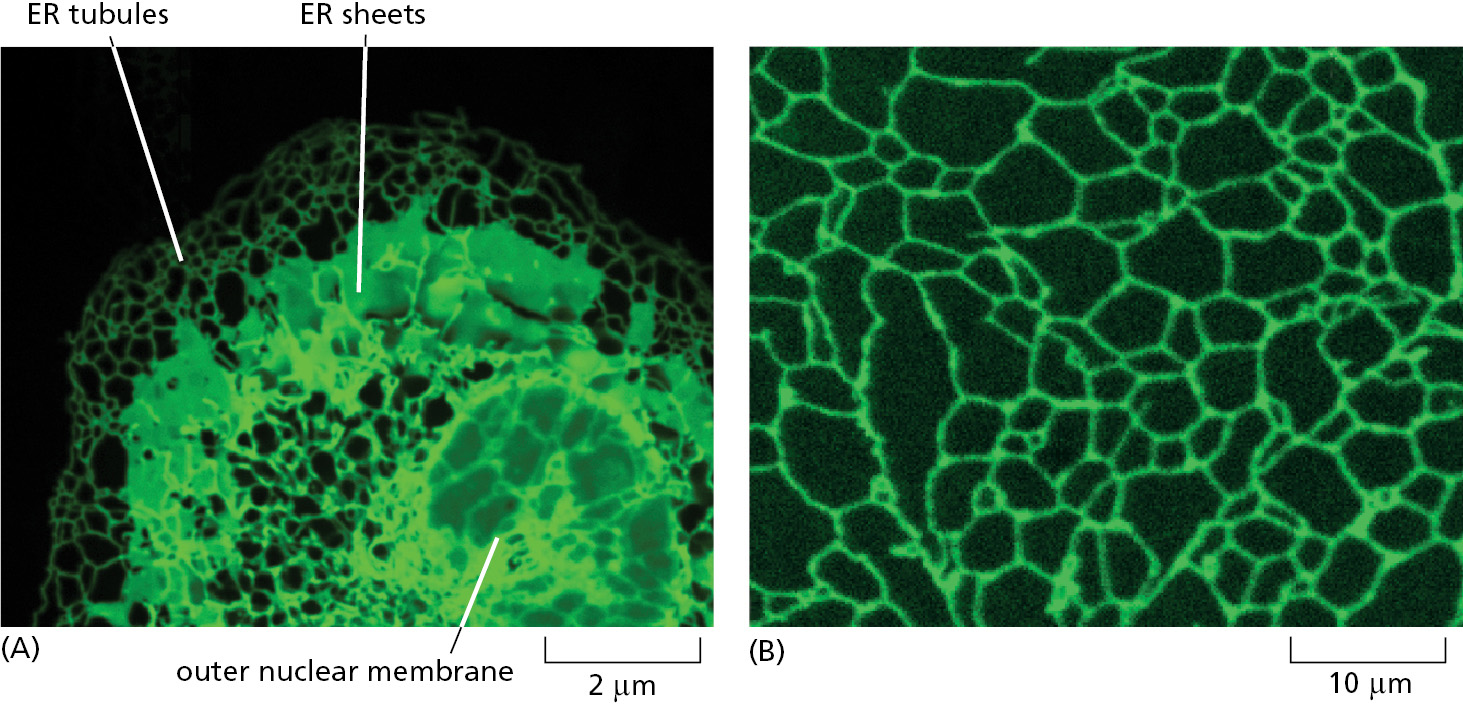
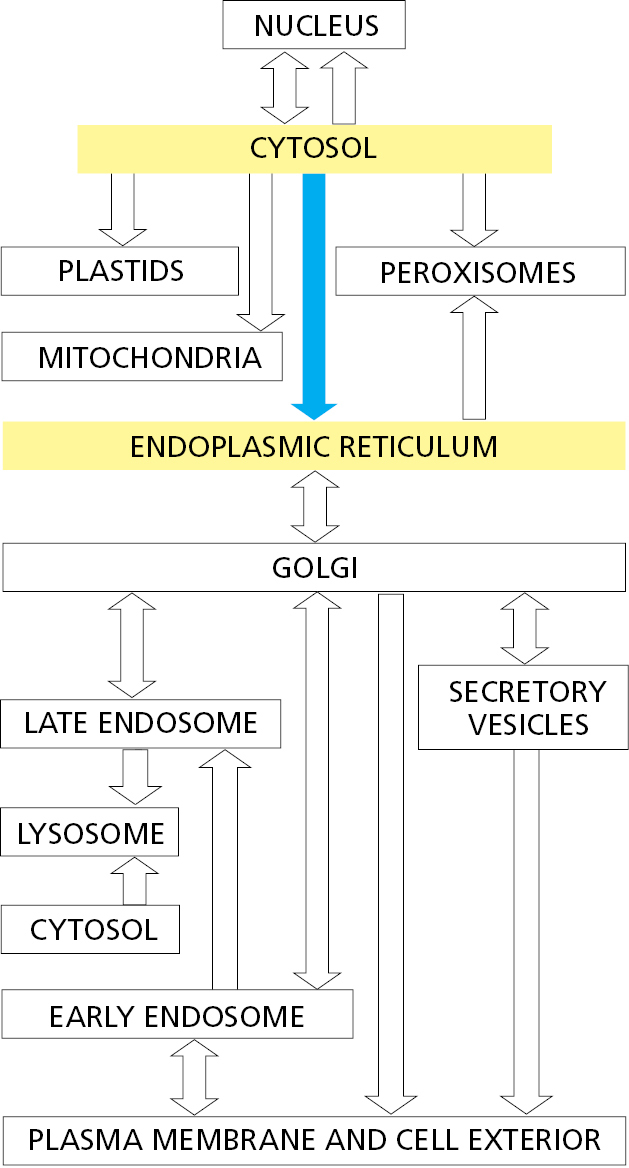
The ER has a central role in the biosynthesis of both lipids and proteins, and the ER lumen stores intracellular Ca2+ that is mobilized in many cell signaling responses (discussed in Chapter 15). The ER membrane is the site of production of many of the transmembrane proteins and lipids of the cell’s organelles, including the ER itself, the Golgi apparatus, lysosomes, endosomes, secretory vesicles, peroxisomes, and the plasma membrane. The ER membrane is also the site at which most of the lipids for mitochondrial and plastid membranes are made. In addition, almost all of the proteins that will be secreted to the cell exterior—plus those destined for the lumen of the ER, Golgi apparatus, or lysosomes—are initially delivered to the ER lumen.
The ER Is Structurally and Functionally Diverse
While the various functions of the ER are essential to every cell, their relative importance varies greatly between individual cell types. To meet different functional demands, distinct regions of the ER become highly specialized. Functional specialization entails dramatic changes in the proportional abundance of different parts of the ER. These changes are observed as characteristically different types of ER membrane in different types of cells. The most visually remarkable specializations are the rough ER and smooth ER (Figure 12–15). The rough appearance is due to the abundance of ribosomes engaged in protein synthesis bound to the surface of this part of the ER. By contrast, regions of smooth ER lack ribosomes and are dedicated to other ER functions such as the biosynthesis and metabolism of lipids. All cells have both rough and smooth ER, but their relative abundance can vary enormously in specialized cells.
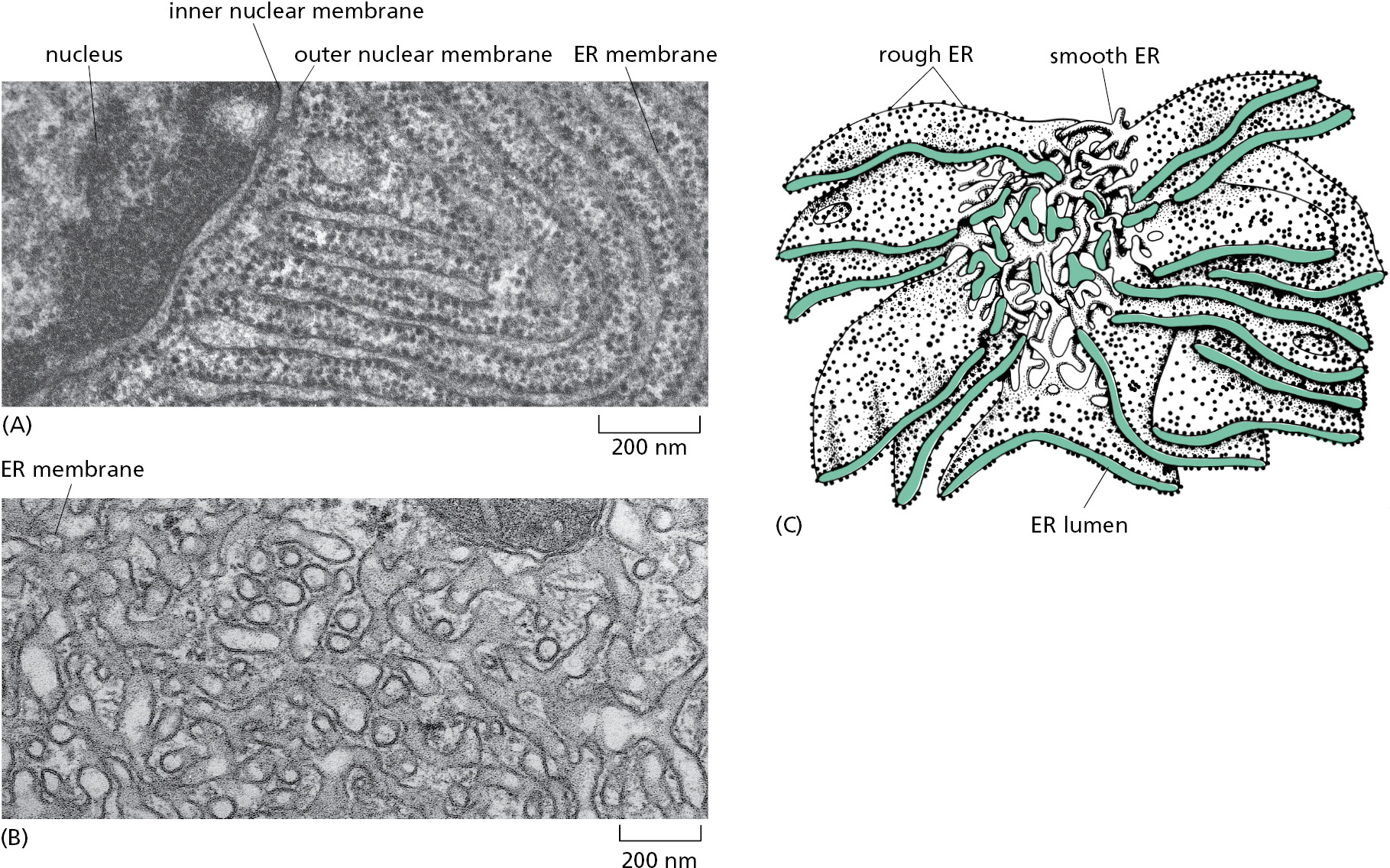
Most secreted proteins are synthesized by the ribosomes that stud the surface of the rough ER. Thus, cells specialized to secrete vast amounts of protein are packed with an abundance of rough ER. For example, exocrine cells of the pancreas secrete their own weight in digestive enzymes every day, explaining why the rough ER makes up 60% of these cells’ membranes (see Table 12–2). Similarly, antibody-secreting plasma cells and insulin-secreting β cells also contain a markedly expanded rough ER. This correlation between highly secretory cells and an abundance of rough ER provided biologists the first clue that the ER is responsible for the synthesis and assembly of secreted proteins.
In contrast to rough ER, functions for the smooth ER are more diverse and can become highly specialized. A type of smooth ER found in all cells is called transitional ER, from which transport vesicles carrying newly synthesized proteins and lipids bud off for transport to the Golgi apparatus. In certain specialized cells, the smooth ER has additional functions that warrant its expansion. For example, cells that synthesize steroid hormones contain prominent smooth ER to accommodate the enzymes that make cholesterol and modify it to form a variety of steroid hormones (see Figure 12–15B).
The main cell type in the liver, the hepatocyte, also has expanded amounts of smooth ER (see Table 12–2) serving two separate purposes. The hepatocyte is the principal site of production of lipoprotein particles, which carry lipids via the bloodstream to other parts of the body. The enzymes that synthesize the lipid components of the particles are enriched in the membrane of the smooth ER. In addition, these membranes contain enzymes that catalyze a series of reactions to detoxify drugs and various harmful compounds produced by metabolism. The most extensively studied of these detoxification reactions are carried out by the cytochrome P450 family of enzymes. They catalyze a series of reactions in which water-insoluble drugs or metabolites that would otherwise accumulate to toxic levels in cell membranes are rendered sufficiently water soluble to leave the cell and be excreted in the urine or bile.
Another crucially important function of the ER in most eukaryotic cells is to sequester Ca2+ from the cytosol. The release of Ca2+ into the cytosol from the ER, and its subsequent reuptake, occur in many rapid responses to extracellular signals, as discussed in Chapter 15. A Ca2+ pump transports Ca2+ from the cytosol into the ER lumen. A high concentration of Ca2+-binding proteins in the ER facilitates Ca2+ storage. In some cell types, specific regions of the ER are specialized for Ca2+ storage. Muscle cells have an abundant, modified smooth ER called the sarcoplasmic reticulum. The release and reuptake of Ca2+ by the sarcoplasmic reticulum trigger myofibril contraction and relaxation, respectively, during each round of muscle contraction (discussed in Chapter 16).
Finally, the smooth ER can be specialized in regions that make intimate contacts with other organelles, most notably the mitochondria, plastids, endosomes, and the plasma membrane (Figure 12–16). These organelle contact sites are enriched for proteins involved in communication or transport of key metabolites between the juxtaposed membranes. For example, the transport of lipids from their site of synthesis in the ER to the mitochondrion is thought to occur at ER–mitochondria contact sites. Contact of ER with the plasma membrane modulates levels of plasma membrane phosphoinositides, which are lipids that participate in numerous signaling pathways (discussed in Chapters 13 and 15). Contacts between other combinations of organelles have also been observed, and it is likely that these are also involved in the selective transfer of lipids and other metabolites.
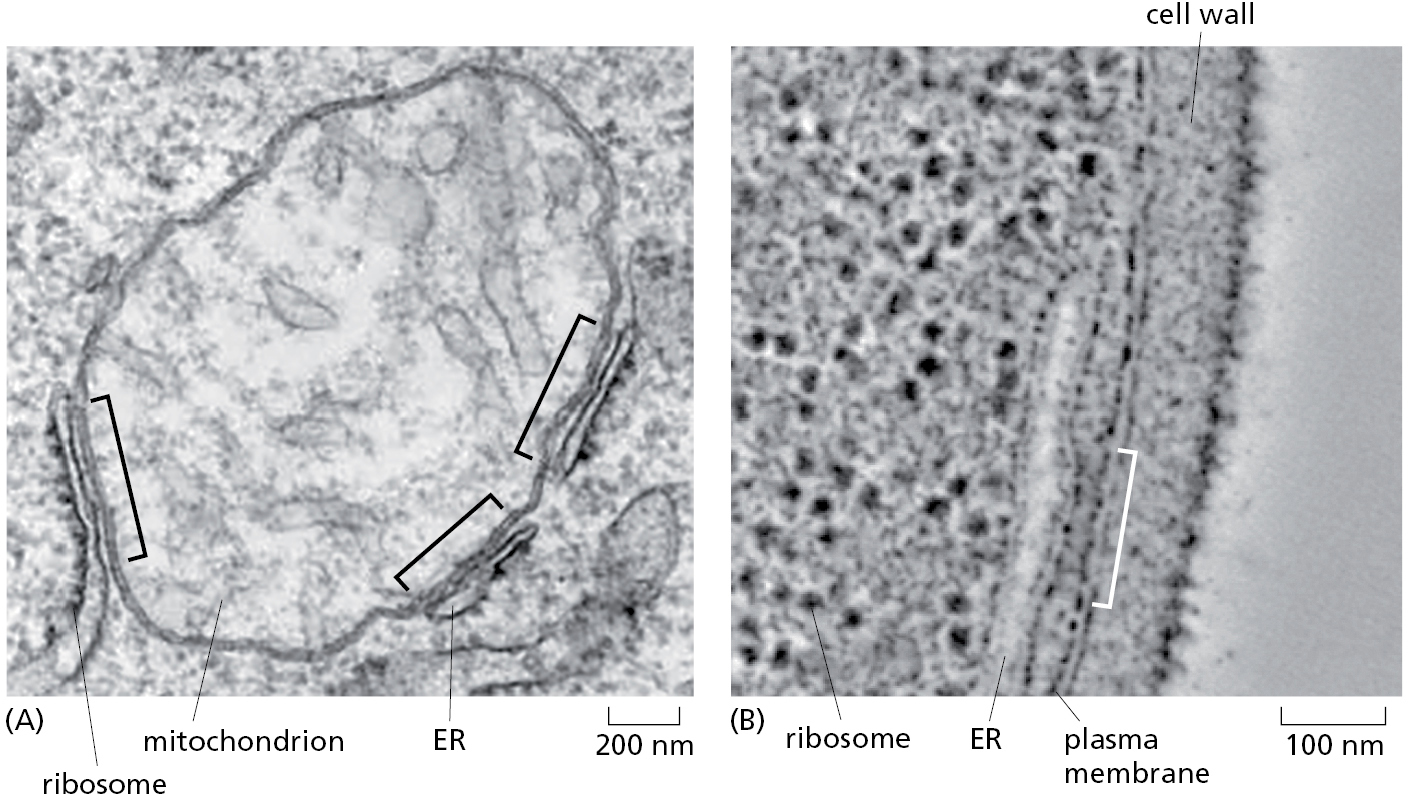
To study the functions and biochemistry of the ER, it is necessary to isolate it. This may seem to be a hopeless task because the ER is intricately interleaved with other components of the cytoplasm. Fortunately, when tissues or cells are disrupted by homogenization, the ER breaks into fragments, which reseal to form small (∼100–200 nm in diameter) closed vesicles called microsomes (Figure 12–17). To the biochemist, microsomes represent small authentic versions of the ER, still capable of protein translocation, protein glycosylation (discussed later), Ca2+ uptake and release, and lipid synthesis. Rough microsomes, derived from rough ER, contain ribosomes on their outside surface and enclose a small part of the ER lumen. Smooth microsomes, which lack ribosomes, are derived from vesiculated fragments of the smooth ER, plasma membrane, Golgi apparatus, endosomes, and mitochondria. The ribosomes attached to rough microsomes make them denser than smooth microsomes. As a result, scientists use equilibrium density centrifugation to separate the rough and smooth microsomes (Figure 12–17). Smooth microsomes derived from different organelles can be further separated on the basis of differences in their protein content. Microsomes have been invaluable in elucidating the molecular aspects of ER function, as we discuss next.
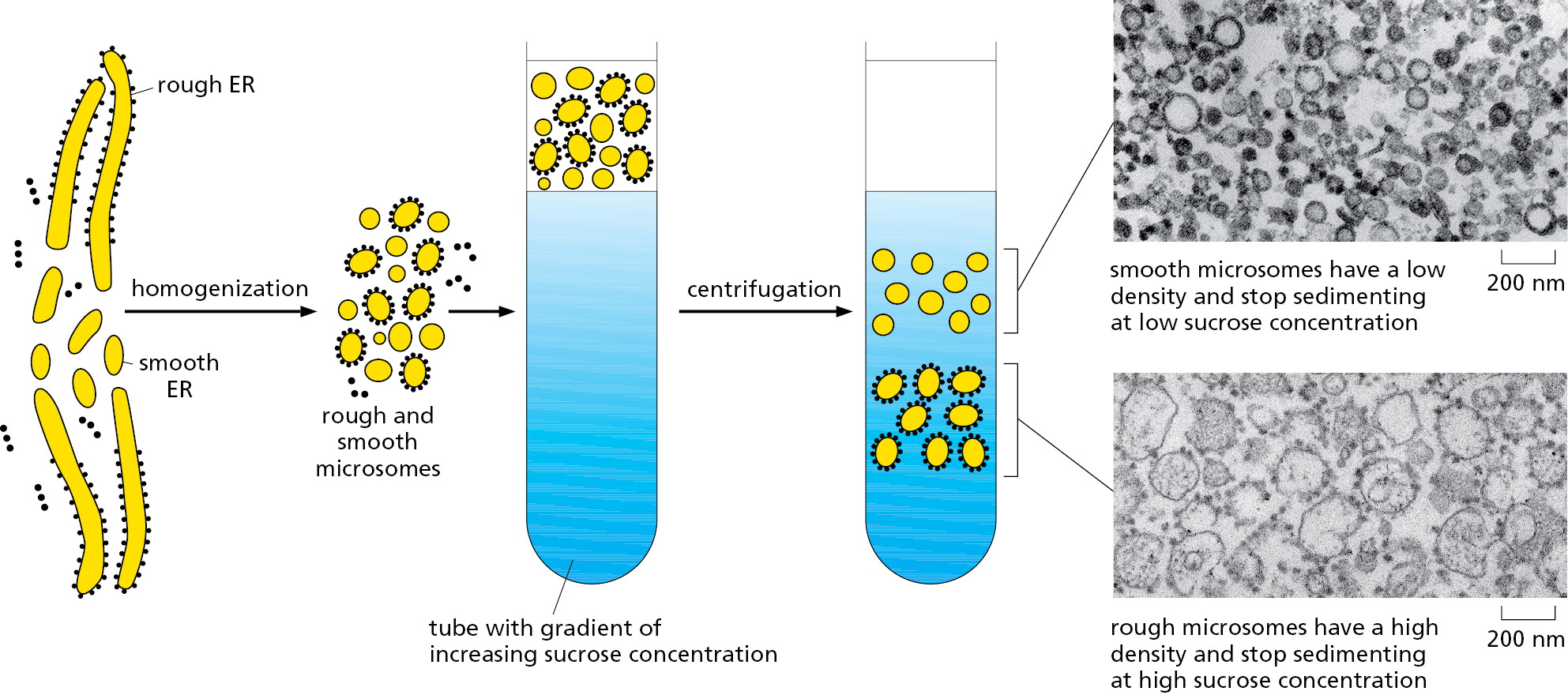
Signal Sequences Were First Discovered in Proteins Imported into the Rough ER
The ER captures selected proteins from the cytosol as they are being synthesized. These proteins are of two types: transmembrane proteins, which become embedded in the ER membrane, and water-soluble proteins, which are fully translocated across the ER membrane into the ER lumen. Some of these proteins function in the ER, but many are destined to reside in another organelle, to reside in the plasma membrane, or to be secreted outside the cell. All of these proteins, regardless of their subsequent fate, are initially directed to the ER membrane by an ER signal sequence.
Signal sequences (and the signal sequence strategy of protein sorting) were discovered in secreted water-soluble proteins that are first translocated across the ER membrane. In the key experiment, the mRNA encoding a secreted protein was added to cytosol extracted from cells. In this cell-free reaction, ribosomes in the cytosol translated the mRNA into a protein that was slightly larger than the normal secreted protein (Figure 12–18). When the reaction was repeated in the presence of microsomes derived from the rough ER, a protein of the correct size was produced and located inside the microsomes (Figure 12–18). By contrast, mRNA encoding a cytosolic protein produced the correctly sized product regardless of the presence or absence of rough microsomes. The signal hypothesis was formulated to explain these observations. According to this model, the mRNA for the secretory protein codes for a protein that is bigger than the protein that is eventually secreted. It was proposed that the extra polypeptide is a signal sequence that directs the secreted protein to the ER membrane. After the signal sequence has served its function, it is cleaved off by a signal peptidase in the ER membrane before the polypeptide chain has been completed.
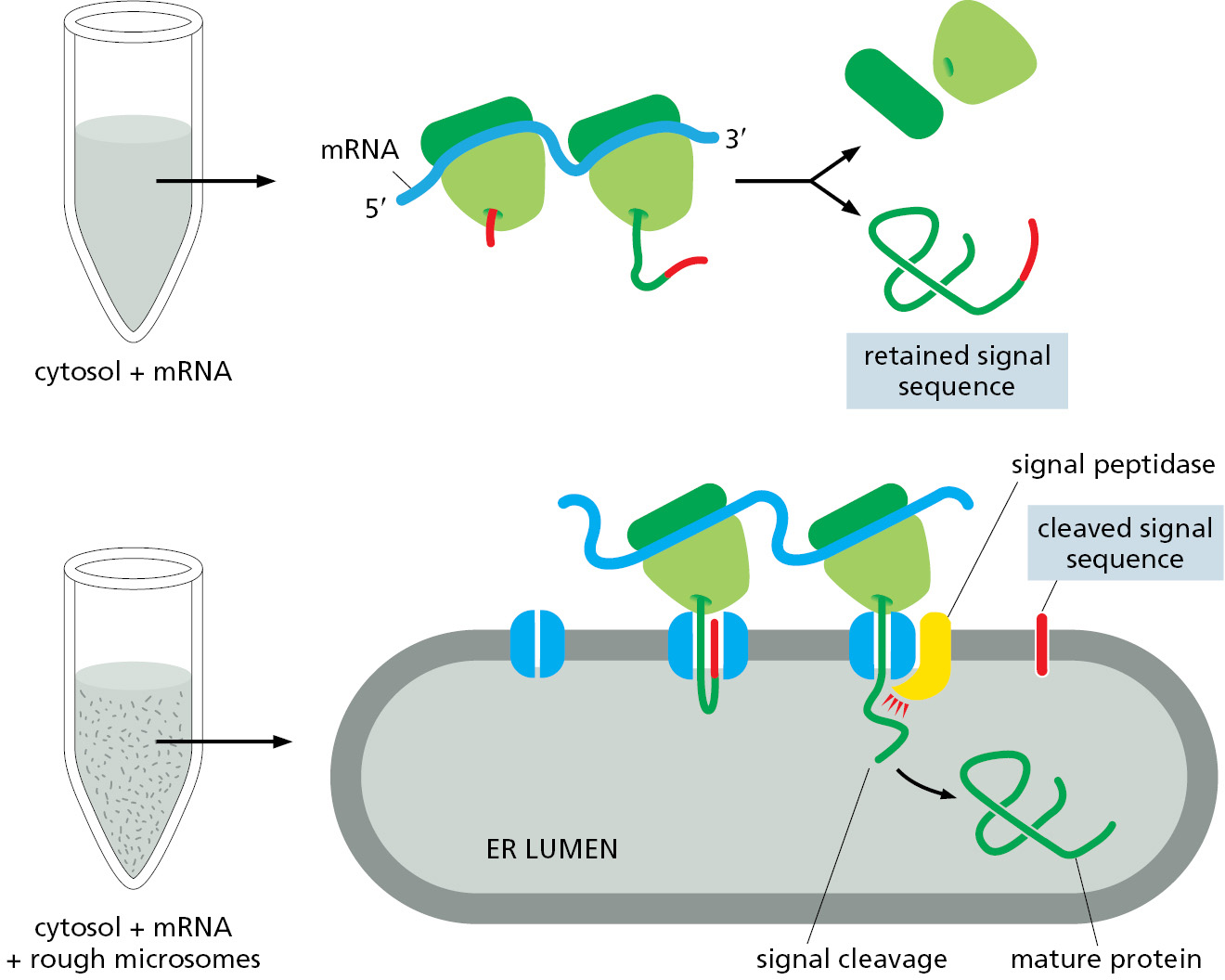
These experiments highlight how a complex cellular process such as ER import can be reconstituted in a cell-free system by mixing together requisite cellular components such as mRNA, cytosol, and microsomes. By combining the constituent parts in different ways, the existence of signal sequences on secreted proteins was deduced long before it became possible to directly sequence their mRNAs. The ease with which this cell-free system could be manipulated proved indispensable for identifying, purifying, and studying the various components of the molecular machinery responsible for ER import. Similar systems were later established to dissect protein transport into and out of the nucleus, protein import into mitochondria and chloroplasts, and vesicular transport.
A Signal-Recognition Particle (SRP) Directs the ER Signal Sequence to a Specific Receptor at the ER
The ER signal sequence is guided to the ER membrane by at least two components: a signal-recognition particle (SRP), which binds to the signal sequence, and an SRP receptor in the ER membrane. SRP is a large complex; in animal cells, it consists of six different polypeptide chains bound to a single RNA molecule (Figure 12–19A). While SRP and SRP receptor have fewer subunits in bacteria, homologs of both components are present in all living organisms. This protein-targeting mechanism therefore arose early in evolution and has been conserved.
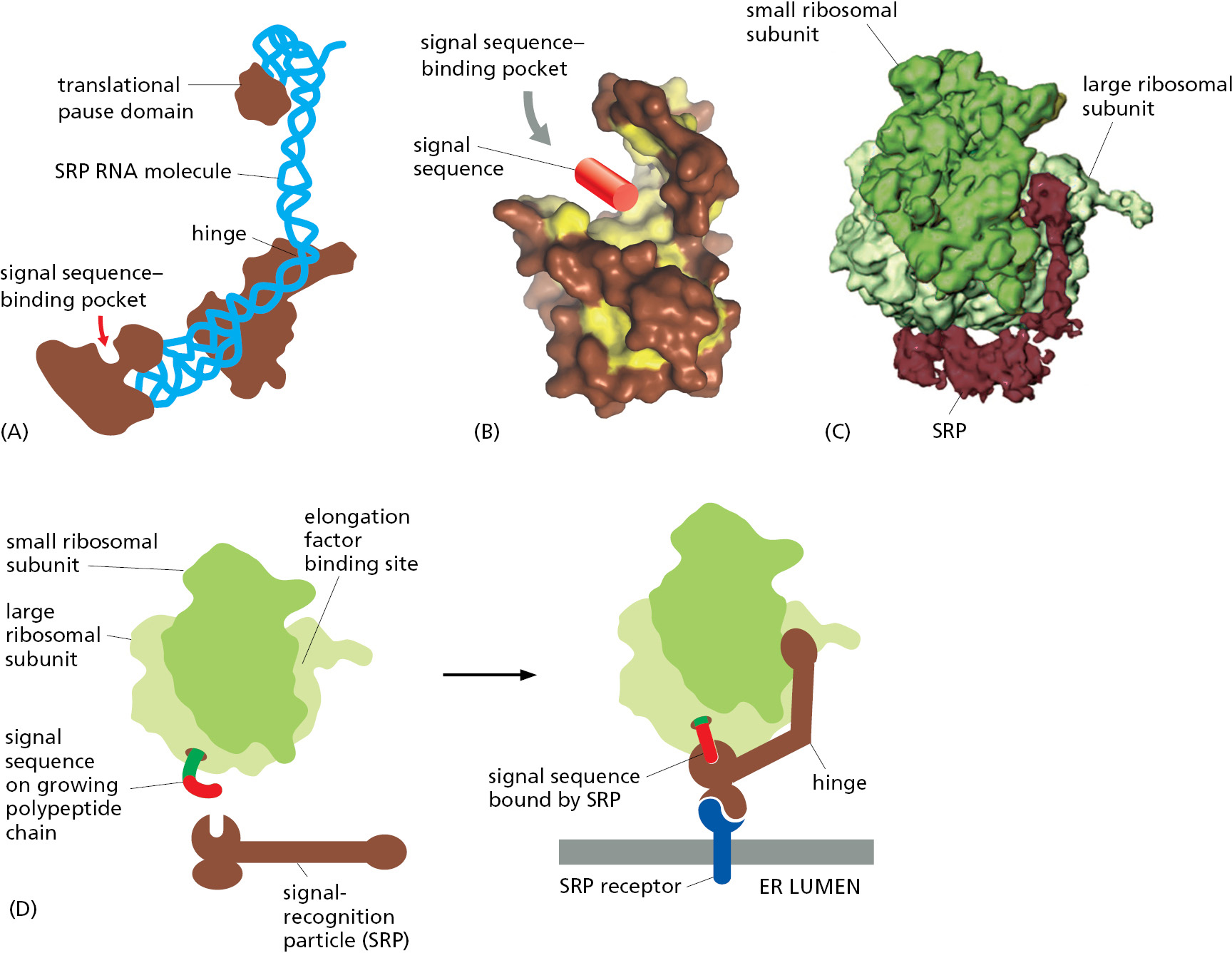
ER signal sequences vary greatly in amino acid sequence, but each has eight or more nonpolar amino acids at its center (see Figure 12–13). How can SRP bind specifically to so many different sequences? The answer has come from structures of one of the SRP proteins, which shows that the signal sequence–binding site is a large hydrophobic pocket enriched in methionines (Figure 12–19B). Because methionines have unbranched, flexible side chains, the pocket is sufficiently plastic to accommodate different hydrophobic signal sequences of various sizes and shapes.
In eukaryotic cells, SRP is a hinged rodlike structure that can wrap along the large ribosomal subunit (Figure 12–19C). The end of SRP that contains the signal sequence–binding pocket is positioned near the ribosomal tunnel through which newly made polypeptides emerge. This allows SRP to engage a signal sequence as it emerges from the ribosome. Once SRP engages a signal sequence, the other end of SRP can bind at the interface between the large and small ribosomal subunits (Figure 12–19D). This is the same site where translation elongation factors bind, so a ribosome engaged by SRP will translate proteins more slowly than normal. Slower translation presumably gives the ribosome enough time to bind to the ER membrane before completion of the polypeptide chain, thereby ensuring that the protein is not released into the cytosol. This safety device may be especially important for secreted and lysosomal hydrolases, which could wreak havoc in the cytosol; cells that secrete large amounts of hydrolases, however, take the added precaution of having high concentrations of hydrolase inhibitors in their cytosol.
When a signal sequence binds, SRP exposes a binding site for an SRP receptor (see Figure 12–19D), which is a transmembrane protein complex in the rough ER membrane. The binding of SRP to its receptor brings the SRP–ribosome complex to an unoccupied protein translocator in the ER membrane. The part of SRP bound near the ribosomal tunnel moves to a different site, allowing the translocator to occupy this position. SRP and SRP receptor are then released, and protein synthesis resumes at full speed. The translocator, which is now tightly bound to the translating ribosome, transfers the growing polypeptide chain across the membrane (Figure 12–20).
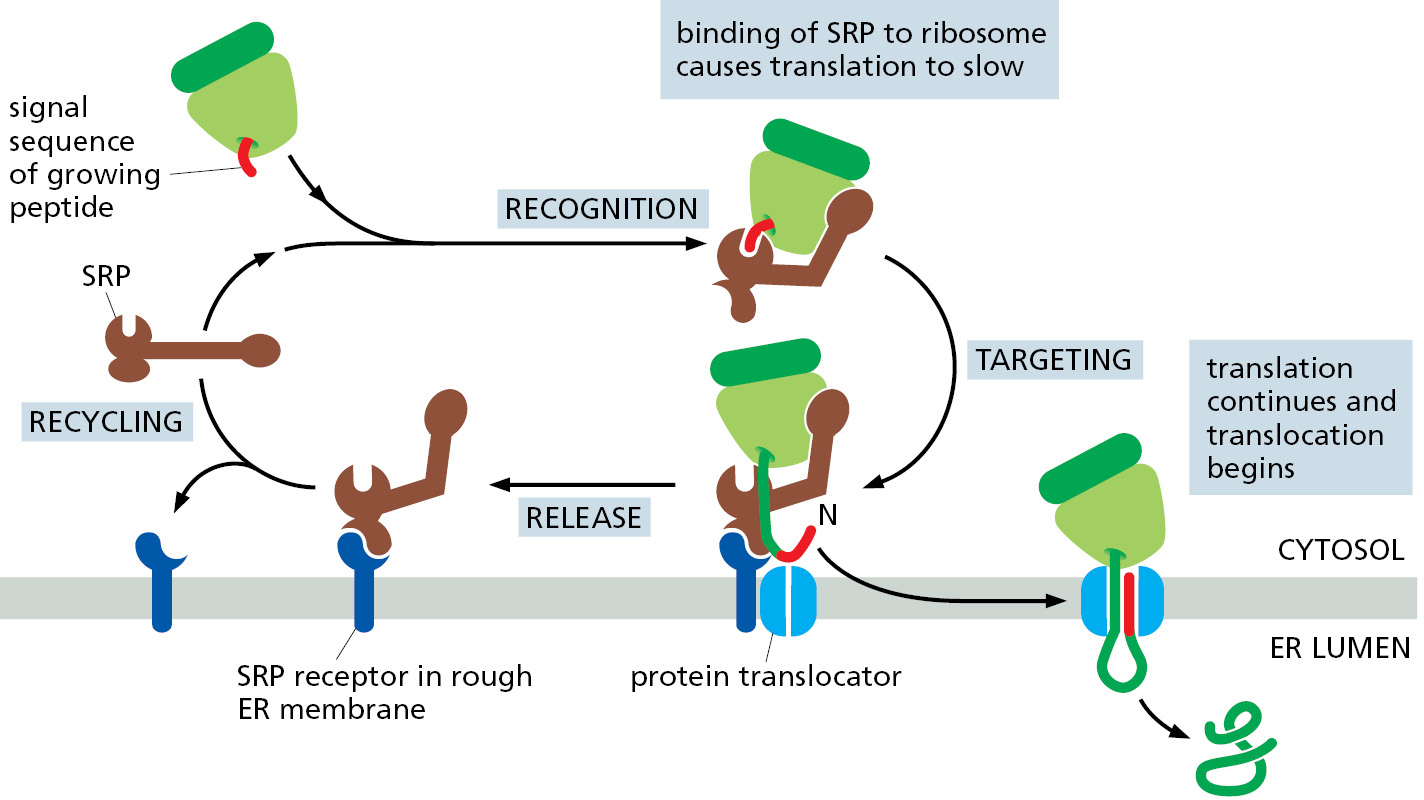
This co-translational transfer process creates two spatially separate populations of ribosomes. Membrane-bound ribosomes, attached to the cytosolic side of the ER membrane, are engaged in the synthesis of proteins that are being concurrently translocated across the ER membrane. Free ribosomes, unattached to any membrane, synthesize all other proteins encoded by the nuclear genome. Membrane-bound and free ribosomes are structurally and functionally identical. They differ only in the proteins they are making at any given time.
Because many ribosomes can engage with a single mRNA molecule, a polyribosome is usually formed. If the mRNA encodes a protein with an ER signal sequence, the polyribosome becomes attached to the ER membrane, directed there by the signal sequences on multiple growing polypeptide chains. The individual ribosomes associated with such an mRNA molecule can return to the cytosol when they finish translation and intermix with the pool of free ribosomes. The mRNA itself, however, remains attached to the ER membrane by a changing population of ribosomes, each transiently held at the membrane by the translocator (Figure 12–21).
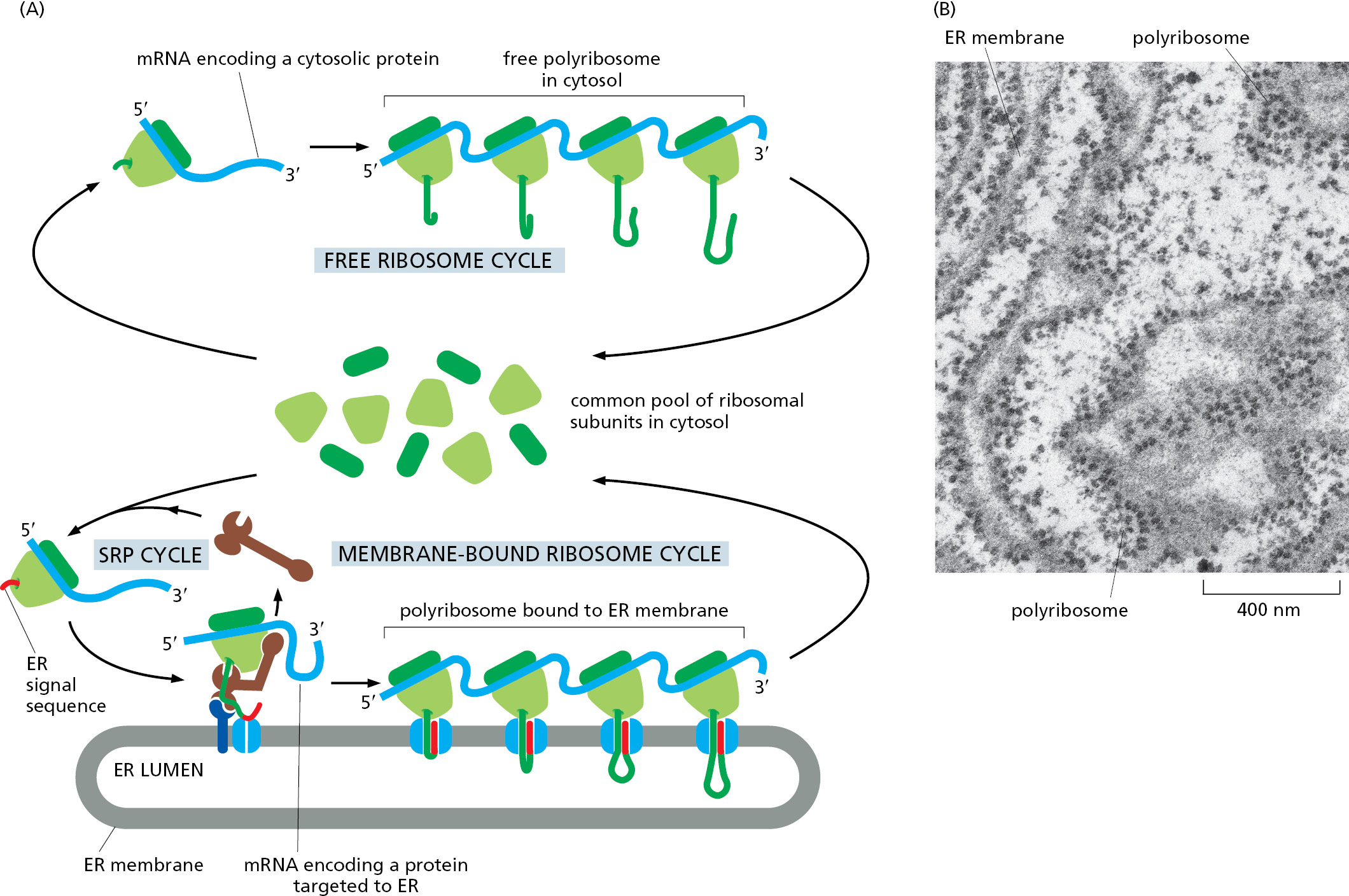
The Polypeptide Chain Passes Through a Signal Sequence–gated Aqueous Channel in the Translocator
It had long been debated whether polypeptide chains are transferred across the ER membrane in direct contact with the lipid bilayer or through a channel in a protein translocator. The debate ended with the identification of the translocator, which was shown to form a water-filled channel across the membrane through which the polypeptide chain passes. The core of the translocator, called the Sec61 complex, is built from three subunits that are highly conserved from bacteria to eukaryotic cells. The structure of the Sec61 translocator revealed that 10 α helices surround a central channel (Figure 12–22). The channel is plugged by a short α helix that keeps the translocator closed when it is idle. It is important to keep the channel closed to prevent ions, such as Ca2+, from leaking out of the ER. During translocation, the plug moves out of the way so the polypeptide can pass through the channel.
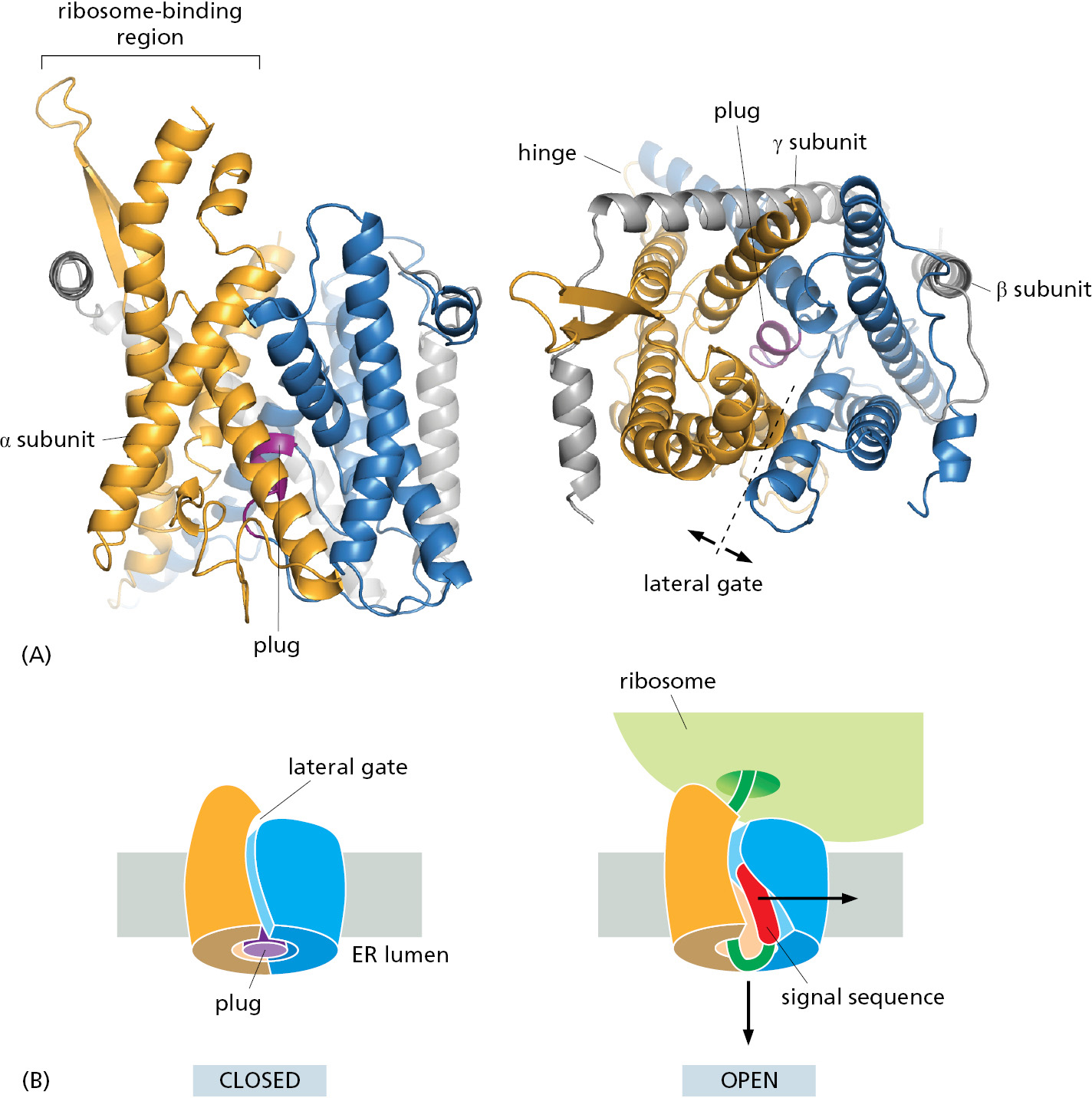
The Sec61 translocator only opens for proteins containing a signal sequence. The ability of the Sec61 translocator to recognize signal sequences provides a proofreading step to ensure that only proteins truly intended for the ER are allowed to enter. Cryo-electron microscopy structures of the Sec61 translocator before and after signal sequence recognition show that the signal sequence wedges into a lateral gate, or seam, in Sec61 with its N-terminus facing the cytosol (Figure 12–23A). Insertion of the signal sequence at this lateral gate widens the central channel and releases the plug. The open translocator then readily accommodates the segment of polypeptide following the signal sequence inside the channel. The signal sequence, which is hydrophobic, laterally exits the gate into the membrane where it is cleaved off by signal peptidase and then rapidly degraded to amino acids by other proteases in the ER membrane and cytosol. As this mechanism illustrates, the lateral gate in the Sec61 translocator provides the access route from Sec61’s central channel into the hydrophobic core of the membrane. In addition to its role in recognition of signal sequences, the lateral gate guides the integration of transmembrane proteins into the ER, as we discuss later.
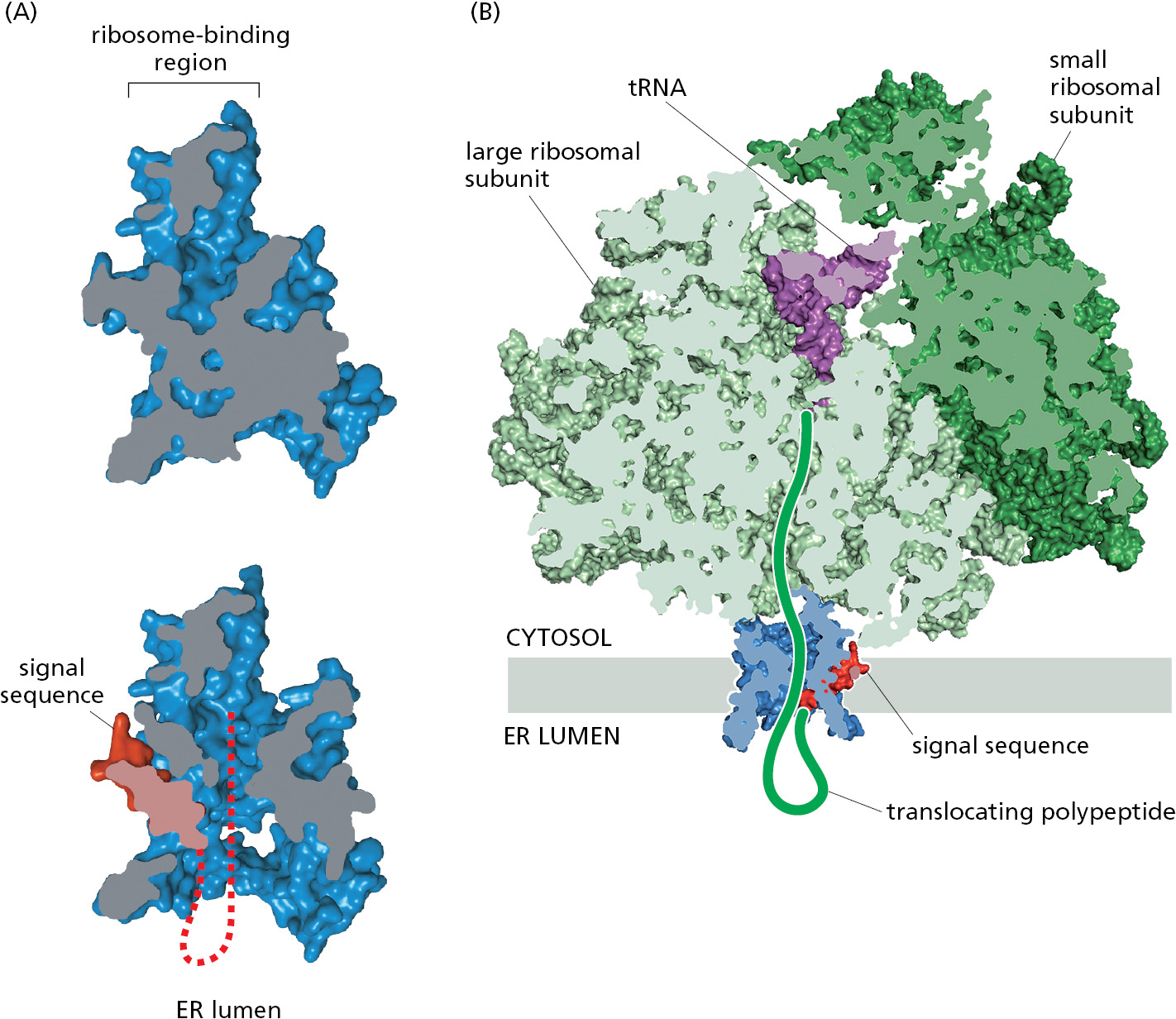
Once the signal sequence has opened the Sec61 translocator and threaded the ensuing polypeptide into the channel, translocation occurs concurrently with continued translation. During translocation, the polypeptide tunnel inside the ribosomal large subunit is aligned with the channel within the Sec61 translocator (Figure 12–23B). This configuration provides a continuous path for the polypeptide from the peptidyl-transferase center in the ribosome, where new amino acids are added to the growing protein chain, to the ER lumen 15 nm away. In this way, the energy used for polypeptide elongation is indirectly harnessed to also drive translocation across the ER membrane.
When translation terminates, the C-terminus of the polypeptide is released from the ribosome and slips through the Sec61 translocator, whose plug returns to close the channel. Thus, the entire process of ER import, from signal sequence recognition by SRP to translocation through the Sec61 translocator, occurs co-translationally before the polypeptide has a chance to fold. This pathway provides one solution to the problem of how to move a large protein across a membrane barrier without leakage of much smaller ions and metabolites during the process.
Translocation Across the ER Membrane Does Not Always Require Ongoing Polypeptide Chain Elongation
Some proteins are completely synthesized in the cytosol as precursors before they are imported into the ER, demonstrating that translocation does not always require ongoing translation (Figure 12–24). This is termed post-translational translocation. Post-translational protein translocation is more common across the yeast ER membrane and the evolutionarily related bacterial plasma membrane. In both cases, the Sec61 translocator (called SecY in bacteria) is used as the translocator; its narrow channel means that precursors can only be translocated as unfolded polypeptides. Thus, precursor proteins do not fold after their initial synthesis in the cytosol. Instead, they interact with other cytosolic proteins that prevent precursor folding or aggregation before they engage the Sec61 translocator. These interacting proteins typically are general chaperone proteins, such as those of the hsp70 family (discussed in Chapter 6), and must dissociate as the unfolded polypeptide is threaded through the translocator.
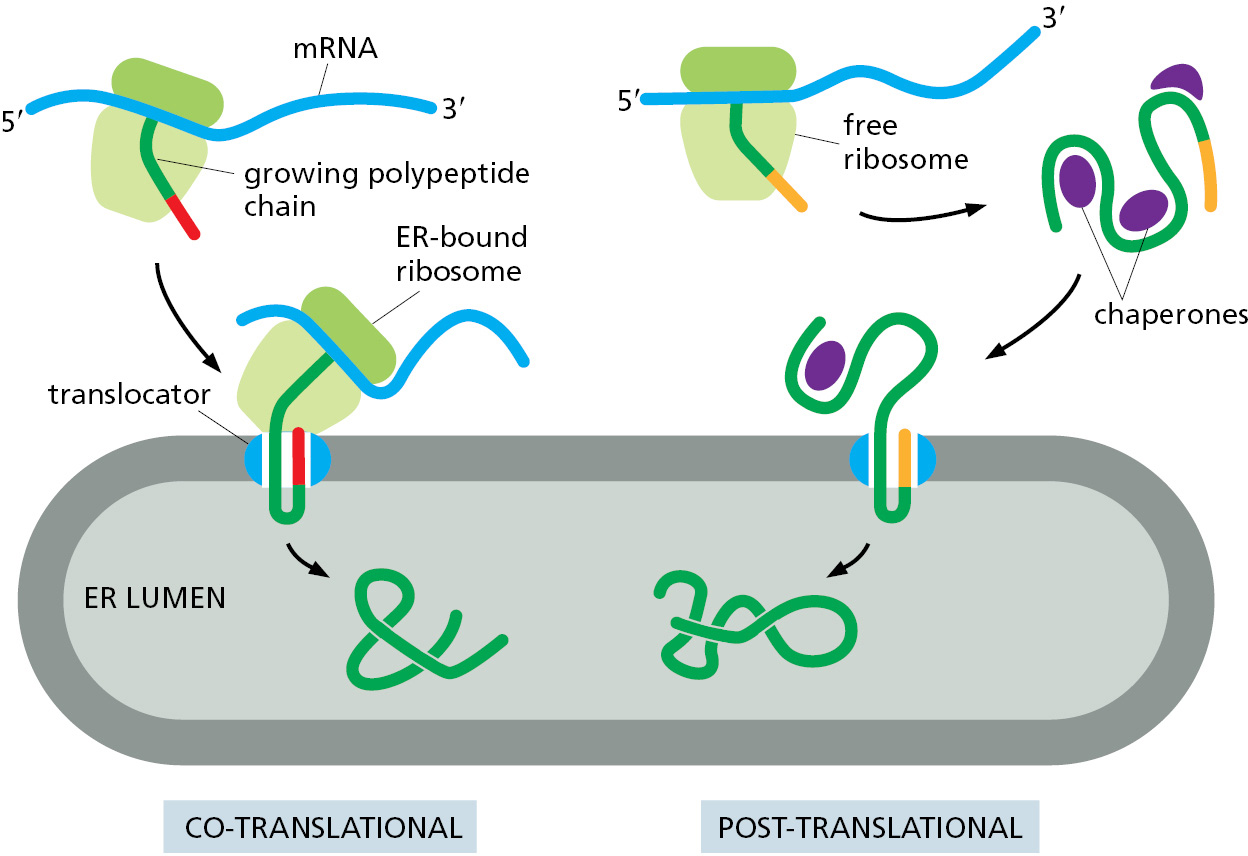
Just as in co-translational translocation discussed earlier, the signal peptide of a precursor directly engages the Sec61 translocator to open the channel. However, the next step of translocation across the membrane occurs differently and relies on accessory proteins that use cellular energy to either pull the polypeptide across the channel from the lumenal side or feed it into the channel from the cytosol (Figure 12–25). To pull proteins into the ER lumen, eukaryotic cells use accessory proteins called Sec62 and Sec63 that associate with the Sec61 translocator and position an hsp70-like chaperone protein (called BiP, for binding protein) adjacent to the lumenal opening of the translocation channel. Like its cytosolic cousin, BiP has a high affinity for unfolded polypeptide chains, and it binds tightly to an imported protein chain as soon as it emerges from the Sec61 translocator in the ER lumen. Tight binding by BiP prevents the protein chain from sliding backwards, favoring more of the chain to emerge into the lumen where it can bind another molecule of BiP. ATP hydrolysis by BiP causes it to release the polypeptide, making it available to bind again to any newly emerged segments of the translocating polypeptide. This energy-driven cycle of binding and release serves as a molecular ratchet that provides the driving force for protein import after a precursor has initially inserted into the Sec61 translocator.
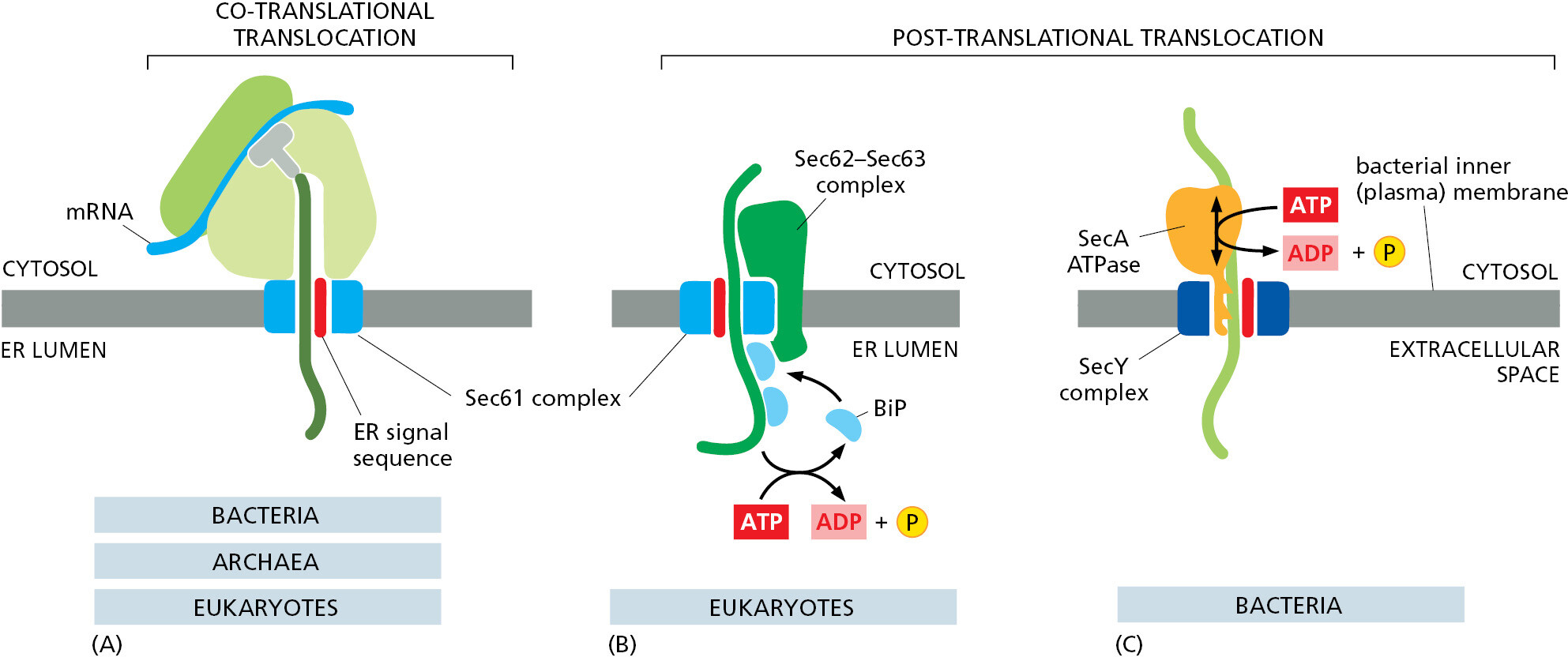
Because bacteria transport proteins directly to the extracellular space, where energy is not available, they use a cytosolic accessory protein called the SecA ATPase. SecA binds to the precursor polypeptide and attaches to the cytosolic side of the translocator, where it undergoes cyclic conformational changes fueled by ATP hydrolysis. Each time an ATP is hydrolyzed, a portion of the SecA protein inserts into the pore of the translocator, pushing a short segment of the precursor protein with it. As a result of this pistonlike ratchet mechanism, the SecA ATPase progressively pushes the polypeptide chain of the transported protein across the membrane.
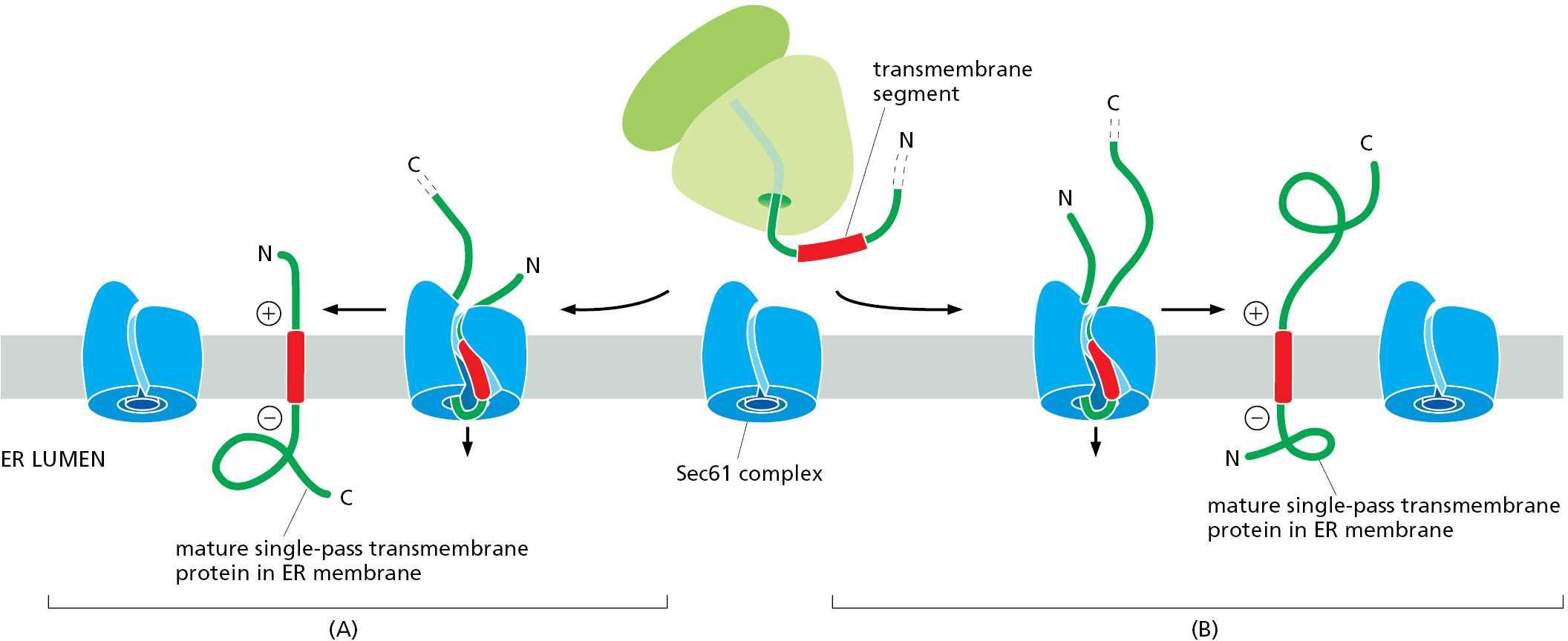
Transmembrane Proteins Contain Hydrophobic Segments That Are Recognized Like Signal Sequences
All of the transmembrane proteins that populate the ER, Golgi apparatus, lysosomes, endosomes, secretory vesicles, and plasma membrane are inserted into the ER membrane before moving to their final destination. Transmembrane proteins made at the ER span the lipid bilayer via one or more α-helical hydrophobic transmembrane segments (see Figure 10–17). Thus, the biosynthesis of membrane proteins requires some parts of the polypeptide chain to be translocated across the lipid bilayer, other parts to remain in the cytosol, and the transmembrane segments to be integrated into the membrane. Despite this additional complexity, the same factors (SRP, SRP receptor, and the Sec61 translocator) just described for transferring a soluble protein into the ER lumen also mediate transmembrane protein integration into the ER membrane. The same factors can be used because the transmembrane segments that define a transmembrane protein resemble the hydrophobic ER signal sequences that direct soluble protein translocation.
In the simplest case, a transmembrane protein contains a single transmembrane segment that will ultimately be embedded in the lipid bilayer as a membrane-spanning α helix. When this transmembrane segment emerges from the ribosome during synthesis, SRP recognizes its hydrophobic α-helical features as a signal sequence and brings this ribosome to the Sec61 translocator at the ER membrane. The transmembrane segment then inserts into the lateral gate of the Sec61 translocator, which is the same site where signal sequences bind. The orientation in which the transmembrane segment inserts into the lateral gate determines whether the protein segment preceding or the one following the transmembrane segment is moved across the membrane into the ER lumen (Figure 12–26). If the N-terminus is short and unfolded, orientation of the transmembrane segment depends on features of the polypeptide chain such as the distribution of nearby charged amino acids and the length of the transmembrane segment. If the preceding N-terminal segment is long and stably folded, it does not cross the membrane through the narrow Sec61 channel. In this case, the C-terminal segment that is still being synthesized, and therefore unfolded, is translocated across the membrane.
Many transmembrane proteins contain large N-terminal lumenal domains. In this case, an N-terminal signal sequence is used to initiate translocation, just as for a soluble protein. In this way, the N-terminus of the mature polypeptide is committed to the ER lumen by the signal sequence, and the remainder of the polypeptide begins translocation through the Sec61 translocator. When a hydrophobic segment in the polypeptide emerges from the ribosome, it inserts into the lateral gate to gain access to the lipid bilayer. Because the hydrophobic segment is more stable in the membrane than in the aqueous channel, it exits the channel laterally, translocation stops, and the rest of the protein is synthesized on the cytosolic side of the ER membrane (Figure 12–27).
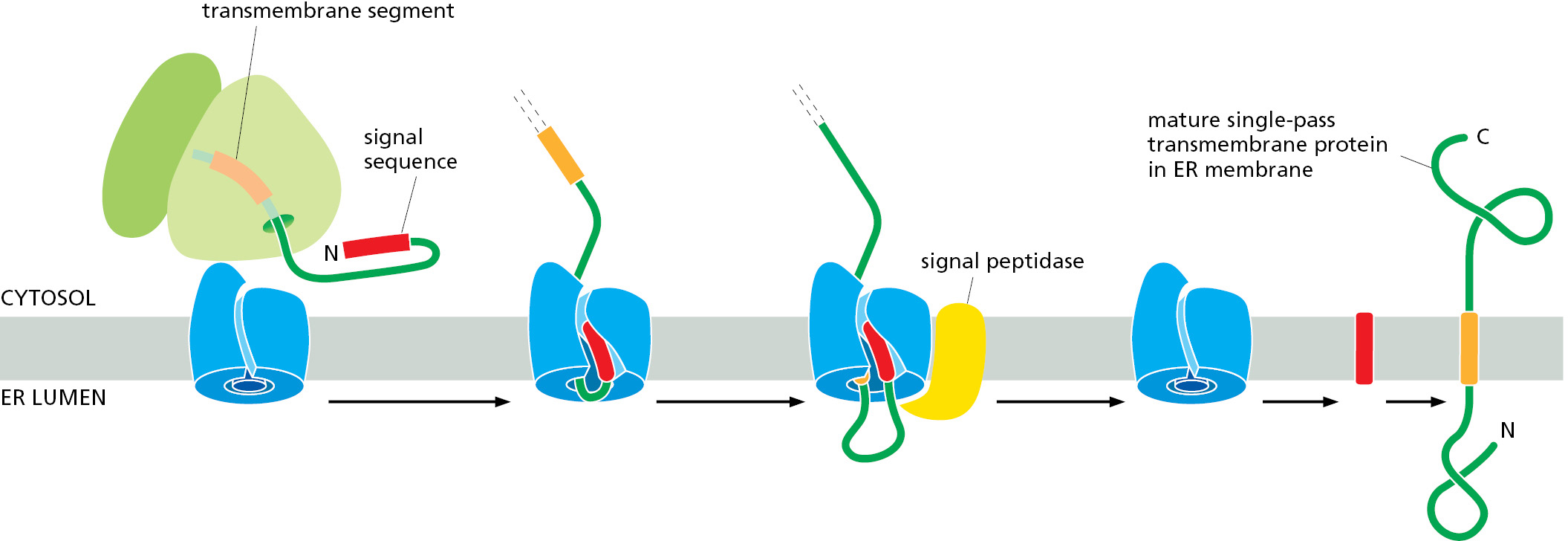
Hydrophobic Segments of Multipass Transmembrane Proteins Are Interpreted Contextually to Determine Their Orientation
In multipass transmembrane proteins, the polypeptide chain passes back and forth repeatedly across the lipid bilayer as hydrophobic α helices (see Figure 10–17). Synthesis of multipass transmembrane proteins up to the first transmembrane segment occurs as we have just described for single-pass transmembrane proteins. Hence, SRP will deliver the protein to the translocator, where the first transmembrane segment will insert into the lateral gate of the Sec61 translocator in an orientation dictated by features of the preceding N-terminal domain and nearby charged amino acids. In this way, insertion of the first transmembrane segment into the membrane effectively locks in the topology for the rest of the protein to come. From this point onward, each successive hydrophobic segment is interpreted by the Sec61 translocator on the basis of the topology and properties of the preceding parts of the protein.
Because of the tight coupling between the ribosome and Sec61 translocator, each hydrophobic segment emerges very close to the lateral gate that provides access to the lipid bilayer. In the simplest cases, the newly emerged hydrophobic segment engages the lateral gate in an orientation opposite to the most recently inserted transmembrane segment and inserts into the lipid bilayer (Figure 12–28). Some transmembrane segments of multipass proteins are only partially hydrophobic and would not be stable in the lipid bilayer on their own. These can nevertheless insert into the membrane if they are able to interact with one of the preceding transmembrane segments that is near the lateral gate of Sec61. This cooperation makes it possible to produce multipass transmembrane proteins that contain hydrophilic parts within the lipid bilayer, which is crucial for many important proteins such as transporters and channels (discussed in Chapter 11). The hydrophilic sequences between the transmembrane segments are either synthesized into the cytosol or threaded through the Sec61 translocator, depending on the orientation of the preceding transmembrane segment. In this way, a multipass protein is woven into the membrane with successive hydrophobic segments achieving opposite orientations until all of them have been inserted into the membrane as transmembrane α helices.
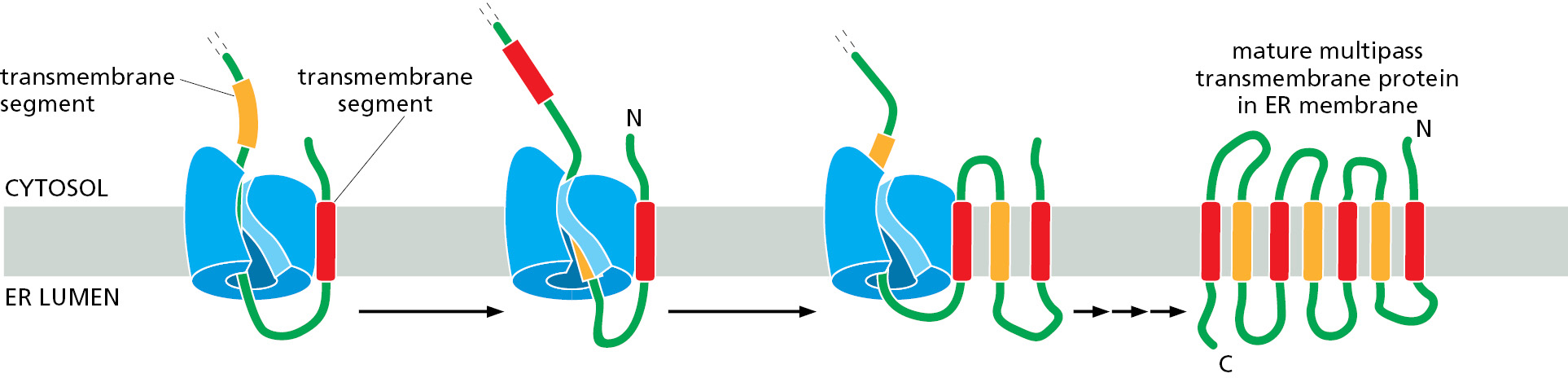
Because membrane proteins are always inserted from the cytosolic side of the ER in this programmed manner, all copies of the same polypeptide chain will have the same orientation in the lipid bilayer. This generates an asymmetrical ER membrane in which the protein domains exposed on one side are different from those exposed on the other side. This asymmetry is maintained during the many membrane budding and fusion events that transport the proteins made in the ER to other cell membranes (discussed in Chapter 13). Thus, the way in which a newly synthesized protein is inserted into the ER membrane determines the orientation of the protein in all of the other membranes as well.
Some Proteins Are Integrated into the ER Membrane by a Post-translational Mechanism
Many important cytosol-facing membrane proteins are anchored in the membrane by a single transmembrane α helix very close to the C-terminus. These tail-anchored proteins include a large number of SNARE protein subunits that guide vesicular traffic (discussed in Chapter 13). When a tail-anchored protein is translated, the ribosome reaches the termination codon while the polypeptide sequence destined to become a transmembrane α helix is still inside the ribosome exit tunnel. Recognition by SRP is therefore not possible, and the protein is released from the ribosome into the cytosol. The hydrophobic segment is recognized by a specialized chaperone complex that transfers it to a targeting factor called Get3 (Figure 12–29). Although unrelated to SRP, Get3 also contains a hydrophobic pocket lined by many methionine side chains to help it recognize diverse hydrophobic segments independent of their exact sequence. Two proteins at the ER membrane called Get1 and Get2 serve not only as the receptor for Get3 but also as the translocator that inserts the hydrophobic segment of the tail-anchored protein into the lipid bilayer. This post-translational targeting mechanism is therefore conceptually similar to SRP-dependent protein targeting (see Figure 12–20). Some tail-anchored proteins are targeted to mitochondria or peroxisomes instead of the ER, but the mechanism of their targeting is not known.
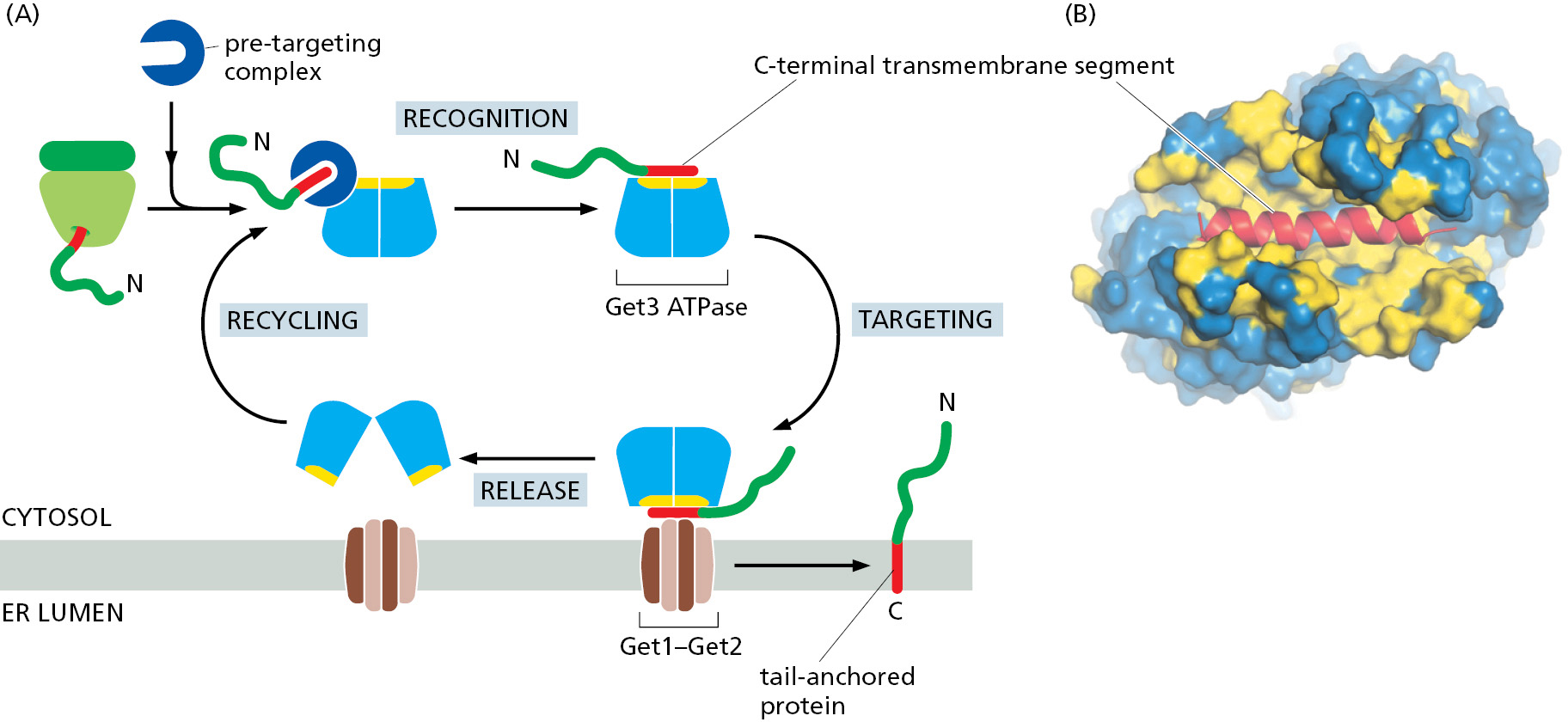
Some Membrane Proteins Acquire a Covalently Attached Glycosylphosphatidylinositol (GPI) Anchor
Another way that proteins are attached to the membrane is by a glycosylphosphatidylinositol (GPI) anchor that is covalently linked to the C-terminus of some proteins destined for the plasma membrane. GPI-anchored proteins are initially made with an N-terminal signal sequence to direct them to the ER and a hydrophobic segment very close to the C-terminus. This hydrophobic segment is selectively recognized by a transamidase enzyme in the ER membrane that simultaneously cleaves off the hydrophobic segment and attaches a preformed GPI anchor to the rest of the protein (Figure 12–30). Many plasma membrane proteins are modified in this way. Because they are attached to the exterior of the plasma membrane only by their GPI anchors, they can be released from cells in soluble form in response to signals that activate a specific phospholipase in the plasma membrane. Trypanosome parasites, for example, use this mechanism to shed their coat of GPI-anchored surface proteins when attacked by the immune system. GPI anchors also participate in directing some plasma membrane proteins into specialized domains, such as lipid rafts, thus laterally segregating them from other membrane proteins (see Figure 10–13).
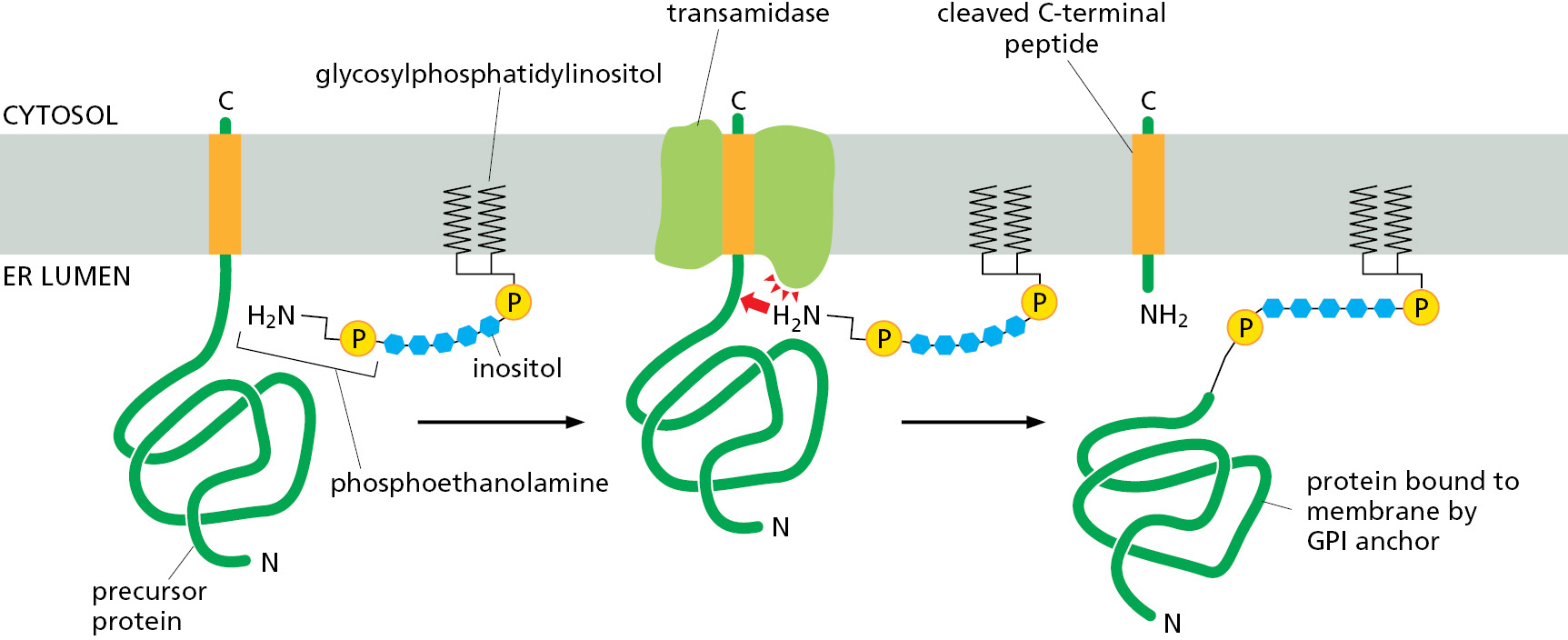
Translocated Polypeptide Chains Fold and Assemble in the Lumen of the Rough ER
Proteins enter the ER lumen as unfolded polypeptides. They must therefore fold and assemble into their correct three-dimensional structures just as newly made proteins in the cytosol must fold (discussed in Chapter 3). To meet this demand, the lumen of the ER contains a high concentration of resident chaperones and other protein-folding catalysts. These ER resident proteins contain an ER retention signal of four amino acids at their C-terminus that is responsible for retaining the protein in the ER (see Figure 12–13; discussed in Chapter 13, p. 768).
The protein BiP, a member of the hsp70 family of chaperone proteins, is a major component of the ER folding machinery. We have already discussed how BiP pulls proteins post-translationally into the ER through the Sec61 ER translocator. Like other chaperones (discussed in Chapter 6), BiP recognizes incorrectly folded proteins, as well as protein subunits that have not yet assembled into their final oligomeric complexes. It does so by binding to exposed hydrophobic amino acid sequences that would normally be buried in the interior of correctly folded or assembled polypeptide chains. The bound BiP both prevents the protein from aggregating and helps keep it in the ER (and thus out of the Golgi apparatus and later parts of the secretory pathway). BiP hydrolyzes ATP to shuttle between high- and low-affinity polypeptide-binding states. In this way, BiP periodically lets go of its substrate proteins to allow them an opportunity to fold, and then re-binds them if folding is not yet achieved.
The ER resident protein protein disulfide isomerase (PDI) catalyzes the oxidation of free sulfhydryl (SH) groups on cysteines to form disulfide (S—S) bonds (Figure 12–31). Almost all cysteines in protein domains exposed to either the extracellular space or the lumen of organelles in the secretory and endocytic pathways are disulfide bonded. Disulfide bonds stabilize the folded state of a protein, enabling it to better withstand a harsh, variable, and chaperone-free extracellular environment. Because proteins often contain multiple cysteines, they sometimes pair incorrectly. PDI resolves this problem by rearranging the disulfide bonds in a protein until it is correctly folded. This is possible because PDI enzymes are capable of operating in reverse to reduce incorrectly paired disulfides of immature proteins. The ER lumen contains multiple members of the PDI family, some of which are specialized for reducing disulfide bonds to fully unfold misfolded proteins that need to be translocated back to the cytosol for degradation (discussed later). All PDI enzymes are therefore oxidoreductases that can catalyze either the formation or breakage of disulfide bonds in their client proteins. The formation of disulfide bonds relies on maintaining an oxidizing environment in the ER lumen. Disulfide bonds form only very rarely in domains exposed to the cytosol because of the reducing environment there.
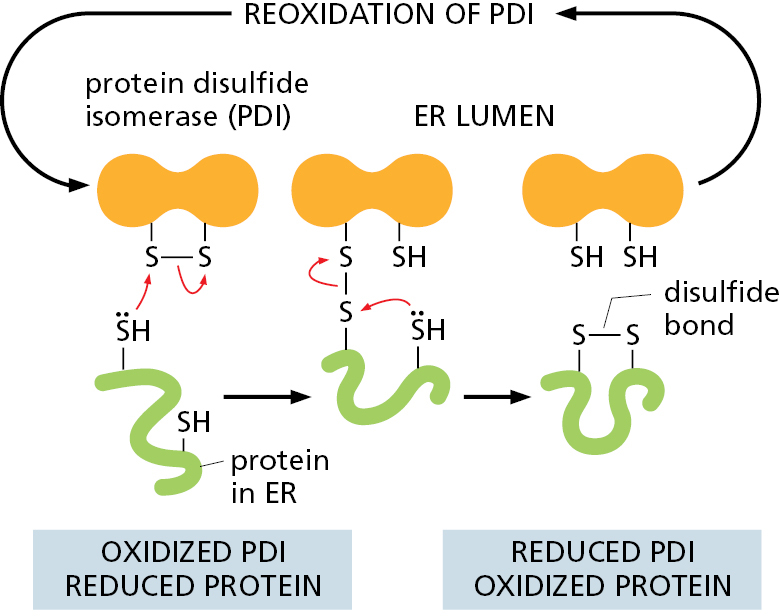
Most Proteins Synthesized in the Rough ER Are Glycosylated by the Addition of a Common N-Linked Oligosaccharide
The covalent addition of oligosaccharides to proteins is one of the major biosynthetic functions of the ER. About half of the soluble and membrane-bound proteins that are processed in the ER—including those destined for transport to the Golgi apparatus, lysosomes, plasma membrane, or extracellular space—are glycoproteins that are modified in this way. Some proteins in the cytosol and nucleus are also glycosylated, but not with large oligosaccharides: they instead carry a much simpler sugar modification, in which a single N-acetylglucosamine group is added to a serine or threonine of the protein.
During the most common form of protein glycosylation in the ER, a preformed precursor oligosaccharide (containing 14 sugars composed of 2 N-acetylglucosamines, 9 mannoses, and 3 glucoses) is transferred as a complete unit to proteins. Because this oligosaccharide is transferred to the side-chain NH2 group of an asparagine in the protein, it is said to be N-linked, or asparagine-linked (Figure 12–32A). A special lipid molecule called dolichol (see Panel 2–5, pp. 102–103) anchors the precursor oligosaccharide in the ER membrane. The precursor oligosaccharide is transferred to the target asparagine in a single enzymatic step by an oligosaccharyl transferase. This membrane-bound enzyme associates with the Sec61 translocator and has its active site exposed on the lumenal side of the ER membrane. This allows the oligosaccharyl transferase to modify newly made proteins immediately after the target asparagine enters the ER lumen during protein translocation (Figure 12–32B).
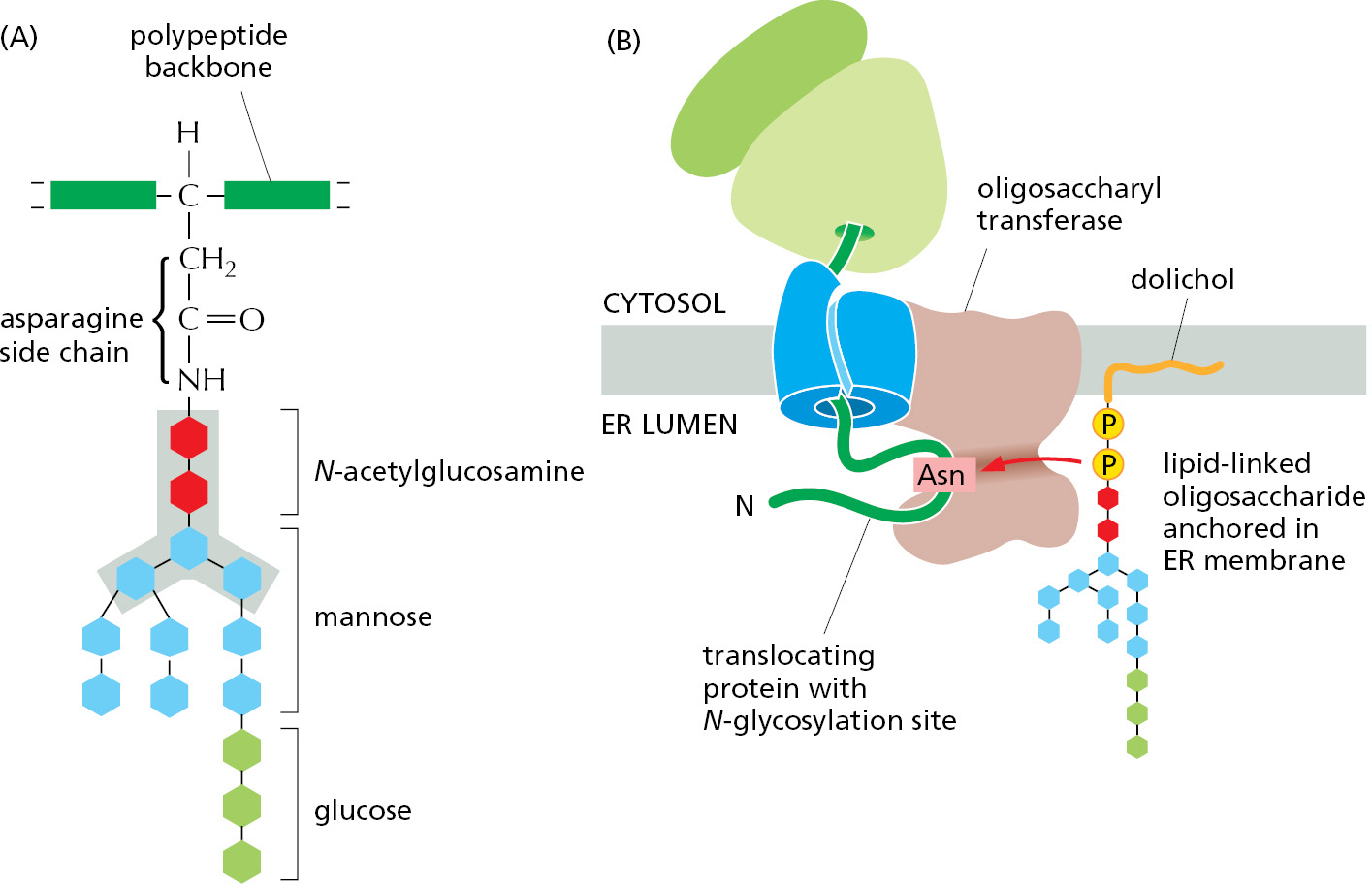
The precursor oligosaccharide is built up sugar by sugar on the membrane-bound dolichol lipid. The sugars are first activated in the cytosol by the formation of nucleotide (UDP or GDP)-sugar intermediates, which then donate their sugar first to the dolichol lipid and then to the partially assembled oligosaccharide tree in an orderly sequence. Partway through this process, the lipid-linked oligosaccharide is flipped, with the help of a transporter, from the cytosolic to the lumenal side of the ER membrane (Figure 12–33).
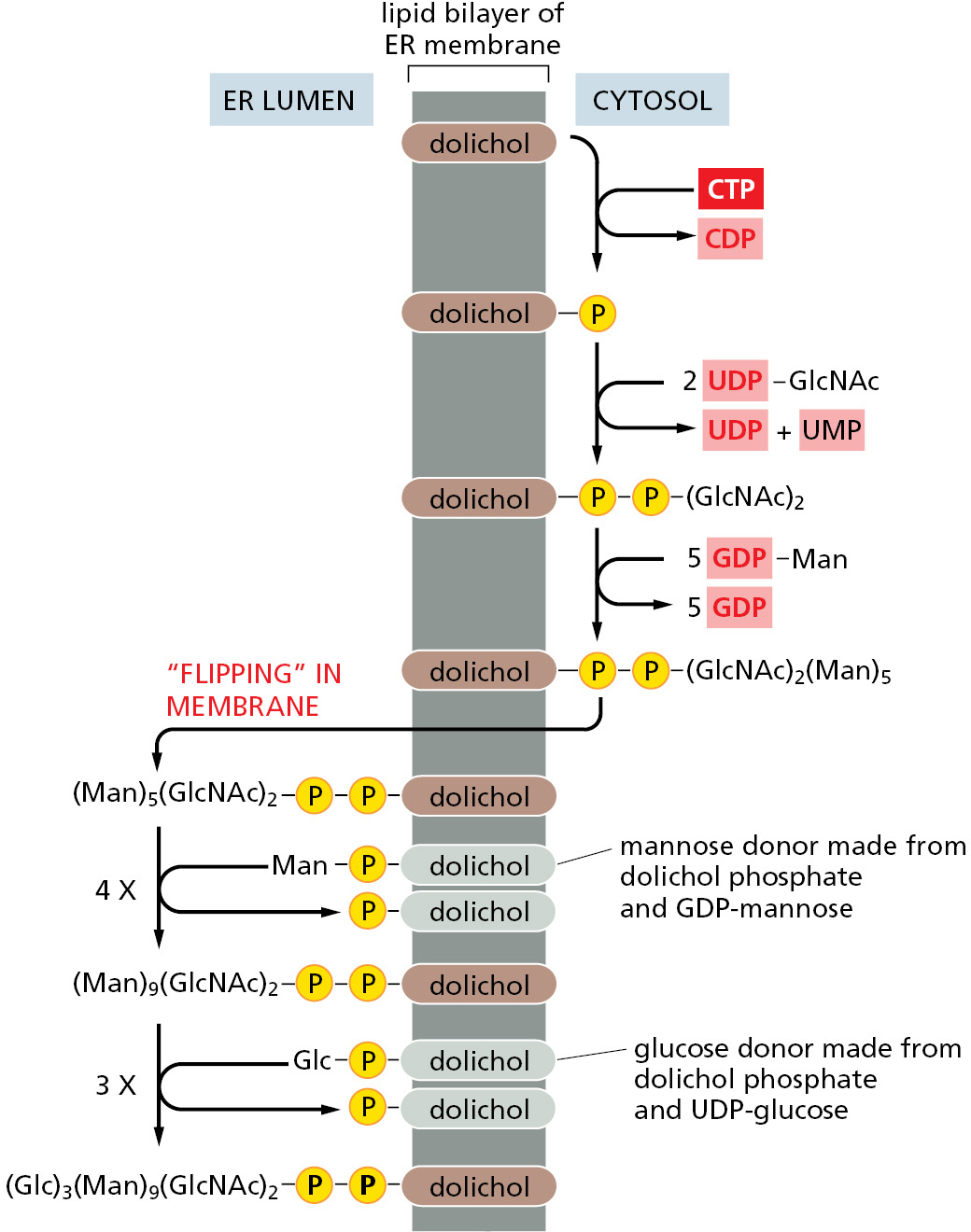
The N-linked oligosaccharides are by far the most common oligosaccharides, being found on 90% of all glycoproteins. Less frequently, oligosaccharides are linked to the hydroxyl group on the side chain of a serine, threonine, hydroxylysine, or hydroxyproline amino acid. The first sugar of these O-linked oligosaccharides is added in the ER. N-linked and O-linked oligosaccharides undergo extensive processing, modification, and extension in the Golgi apparatus (Chapter 13), producing the diversity of oligosaccharide structures observed on mature glycoproteins.
Oligosaccharides Are Used as Tags to Mark the State of Protein Folding
It has long been debated why glycosylation is such a common modification of proteins that enter the ER. One particularly puzzling observation has been that some proteins require N-linked glycosylation for proper folding in the ER, yet the precise location of the oligosaccharides attached to the protein’s surface does not seem to matter. A clue to the role of glycosylation in protein folding came from studies of two ER chaperone proteins, which are called calnexin and calreticulin because they require Ca2+ for their activities. These chaperones are carbohydrate-binding proteins, or lectins, which bind to oligosaccharides on incompletely folded proteins and retain them in the ER. Like other chaperones, they prevent incompletely folded proteins from irreversibly aggregating. Both calnexin and calreticulin also promote the association of incompletely folded proteins with another ER chaperone, which binds to cysteines that have not yet formed disulfide bonds.
How do calnexin and calreticulin distinguish properly folded from incompletely folded proteins? The answer lies in the structure of the oligosaccharide attached to the protein. Shortly after a newly made protein acquires an N-linked precursor oligosaccharide, ER glucosidases rapidly remove two glucoses, leaving behind a single terminal glucose. This singly glucosylated oligosaccharide is recognized by calnexin and calreticulin, ensuring that all newly made (and hence, likely to be not yet folded) glycoproteins bind to one of these chaperones. This last glucose is removed over time, leaving a de-glucosylated glycoprotein that no longer binds to calnexin or calreticulin. If the glycoprotein is folded, it can leave the ER. However, yet another ER enzyme, a glucosyl transferase, re-adds the terminal glucose selectively to glycoproteins that have not yet folded completely. The terminal glucose then causes re-association of the unfolded protein with calnexin or calreticulin. Thus, glucose trimming (by glucosidases) and glucose addition (by the glucosyl transferase) drive cycles of dissociation and re-association with calnexin and calreticulin until a newly made unfolded protein has achieved its fully folded state (Figure 12–34).
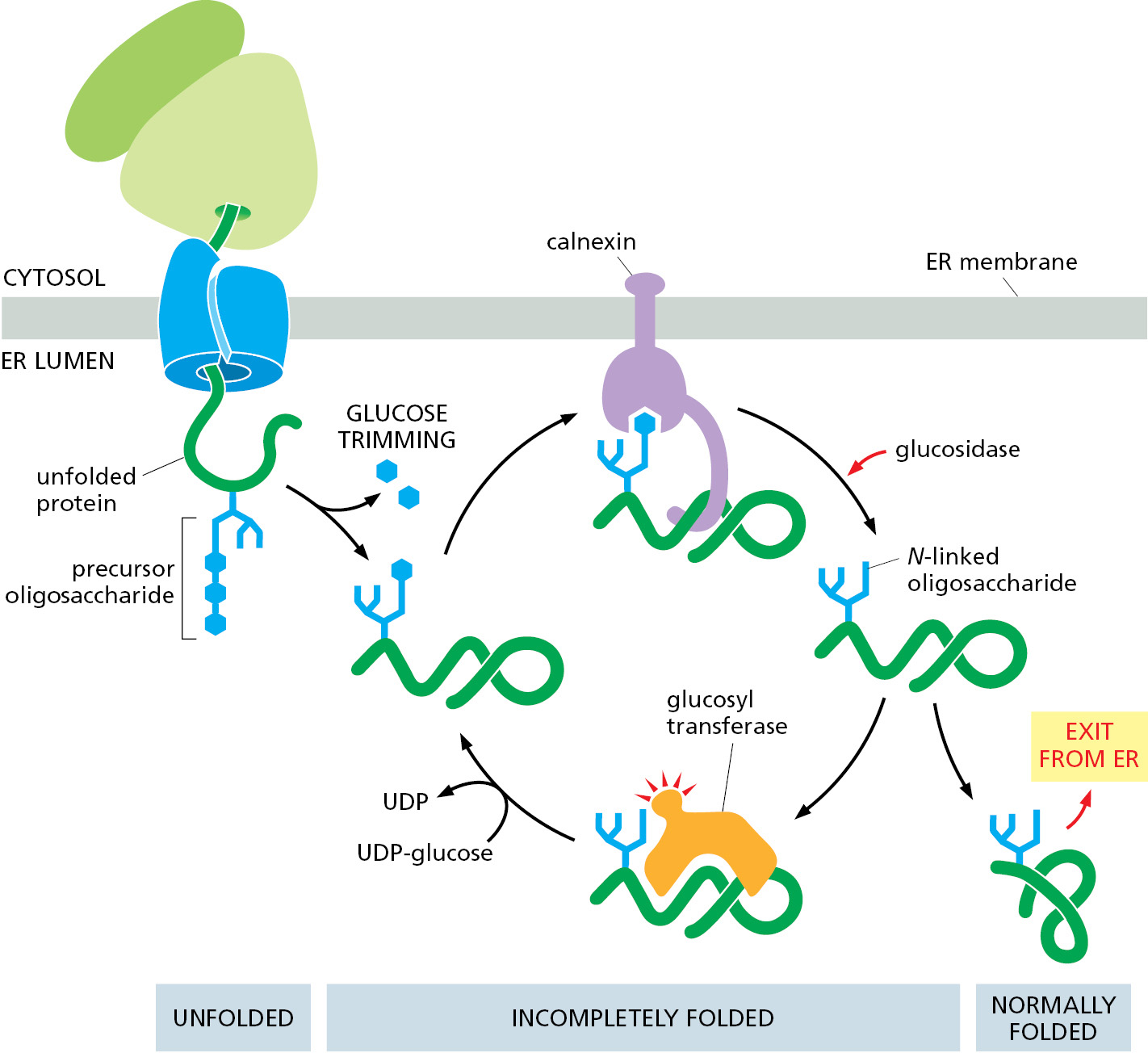
Improperly Folded Proteins Are Exported from the ER and Degraded in the Cytosol
Despite all the help from chaperones, many protein molecules translocated into the ER fail to achieve their properly folded or oligomeric state. Such proteins are exported from the ER back into the cytosol, where they are degraded in proteasomes (discussed in Chapter 6). In many ways, the mechanism of such retrotranslocation is similar to other post-translational modes of translocation. For example, like post-translational import into the ER, chaperone proteins are necessary to keep the polypeptide chain in an unfolded state prior to and during translocation. Similarly, a source of energy is required to provide directionality to the transport and to pull the protein into the cytosol. Finally, a translocator is necessary.
Selecting proteins from the ER for degradation is a challenging process: misfolded proteins or unassembled protein subunits should be degraded, but folding intermediates of newly made proteins should not. Help in making this distinction comes from the N-linked oligosaccharides, which serve as timers that measure how long a protein has spent in the ER. The slow trimming of a particular mannose on the core oligosaccharide tree by an enzyme (a mannosidase) in the ER creates a new oligosaccharide structure that ER-lumenal lectins of the retrotranslocation apparatus recognize. A protein that folds and exits from the ER faster than the mannosidase can remove its target mannose therefore escapes degradation.
In addition to the lectins in the ER that recognize the oligosaccharides, chaperones and protein disulfide isomerases associate with the proteins that must be degraded. The chaperones prevent the unfolded proteins from aggregating, and the disulfide isomerases break disulfide bonds that may have formed incorrectly, so that a linear polypeptide chain can be translocated back into the cytosol.
Multiple translocator complexes move different proteins from the ER membrane or lumen into the cytosol. Translocator complexes always contain an E3 ubiquitin ligase enzyme (Chapter 6), which attaches polyubiquitin tags to the unfolded proteins as they emerge into the cytosol, marking them for destruction. Fueled by the energy derived from ATP hydrolysis, a hexameric ATPase of the family of AAA-ATPases (see Figure 6–88) pulls the unfolded protein through the translocator into the cytosol. An N-glycanase removes en bloc any oligosaccharide chains attached to the retrotranslocated protein. Guided by its ubiquitin tag, the de-glycosylated polypeptide is rapidly fed into proteasomes, where it is degraded (Figure 12–35).
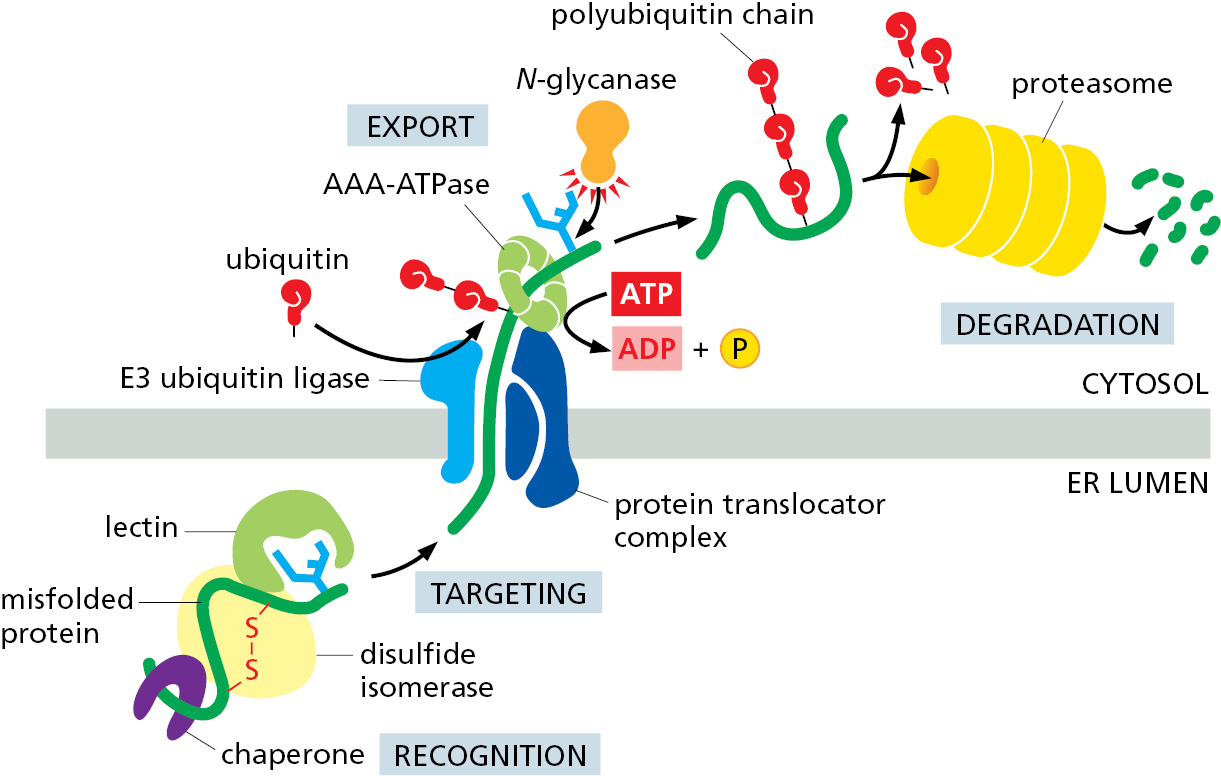
Misfolded Proteins in the ER Activate an Unfolded Protein Response
Cells carefully monitor the amount of misfolded protein in various compartments. An accumulation of misfolded proteins in the cytosol, for example, triggers a heat-shock response (discussed in Chapter 6), which stimulates the transcription of genes encoding cytosolic chaperones that help to refold the proteins. Similarly, an accumulation of misfolded proteins in the ER triggers an unfolded protein response, which stimulates transcription of genes that collectively improve the protein-folding capacity of the ER. The stimulated genes code for ER chaperones, the machinery for protein retrotranslocation and degradation, factors for protein transport out of the ER, and factors for expansion of the ER. This multipronged response operates by coupling the detection of misfolded proteins in the ER lumen to the production of transcription regulatory proteins that enter the nucleus to tune the transcription of hundreds of genes.
How do misfolded proteins in the ER signal to the nucleus? There are three parallel pathways that execute the unfolded protein response (Figure 12–36). The first pathway, which was initially discovered in yeast cells, is conserved in all eukaryotic cells and is particularly remarkable. Misfolded proteins in the ER cause IRE1, a transmembrane protein kinase in the ER, to oligomerize and phosphorylate itself. This mechanism of activation is similar to how some cell-surface receptor kinases in the plasma membrane are activated (discussed in Chapter 15). Oligomeric and phosphorylated IRE1 enables its cytosolic endoribonuclease domain to remove an intron from a specific cytosolic mRNA molecule. IRE1 accomplishes this task by cleaving the mRNA at two positions that are then joined together by an RNA ligase. The mRNA produced by this splicing reaction is translated to produce an active transcription regulatory protein that increases expression of a subset of the genes of the unfolded protein response (Figure 12–37). The regulated splicing of a cytosolic mRNA by IRE1 is a unique exception to the rule that all mRNA splicing occurs in the nucleus and is catalyzed by the spliceosome.
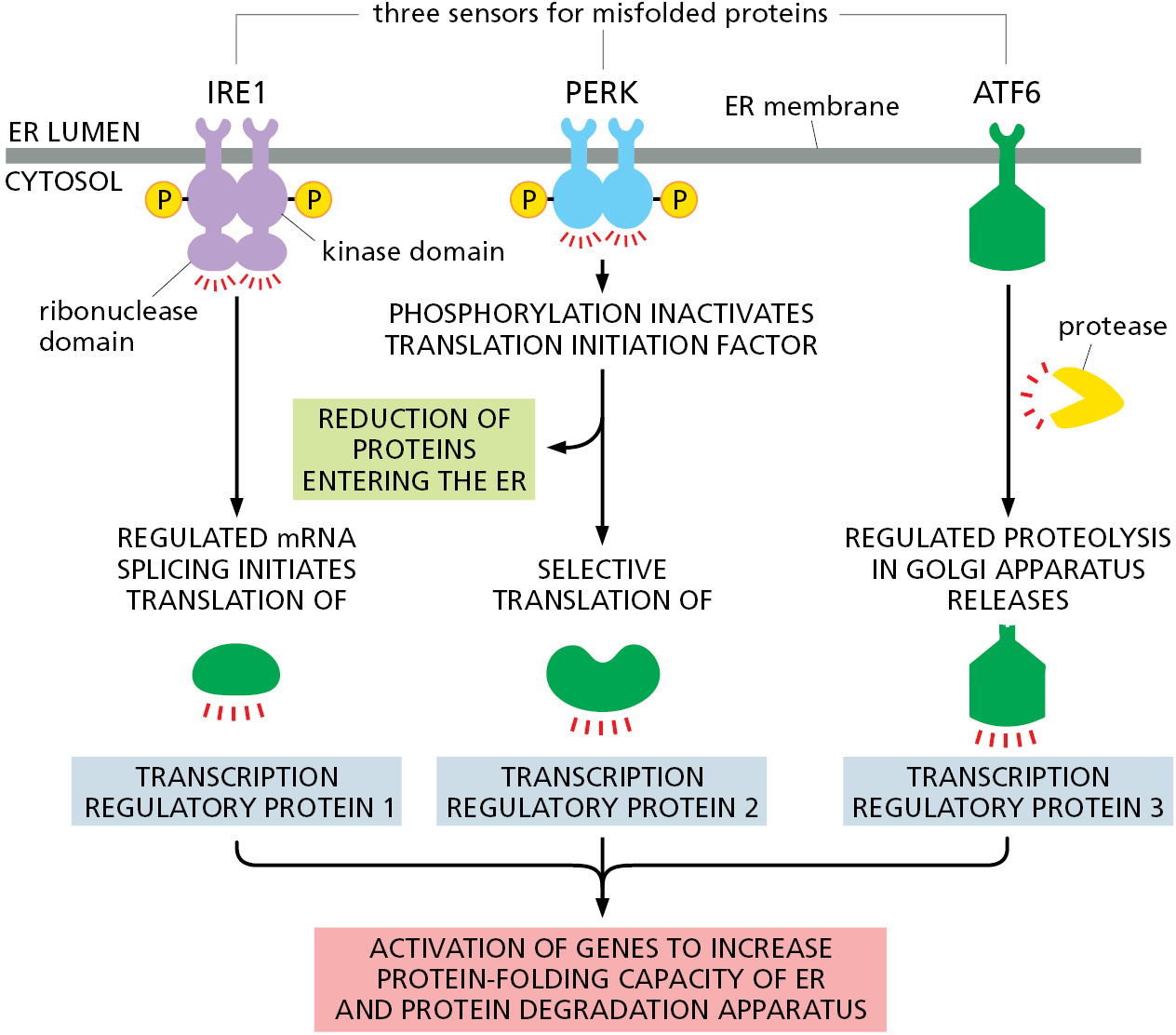
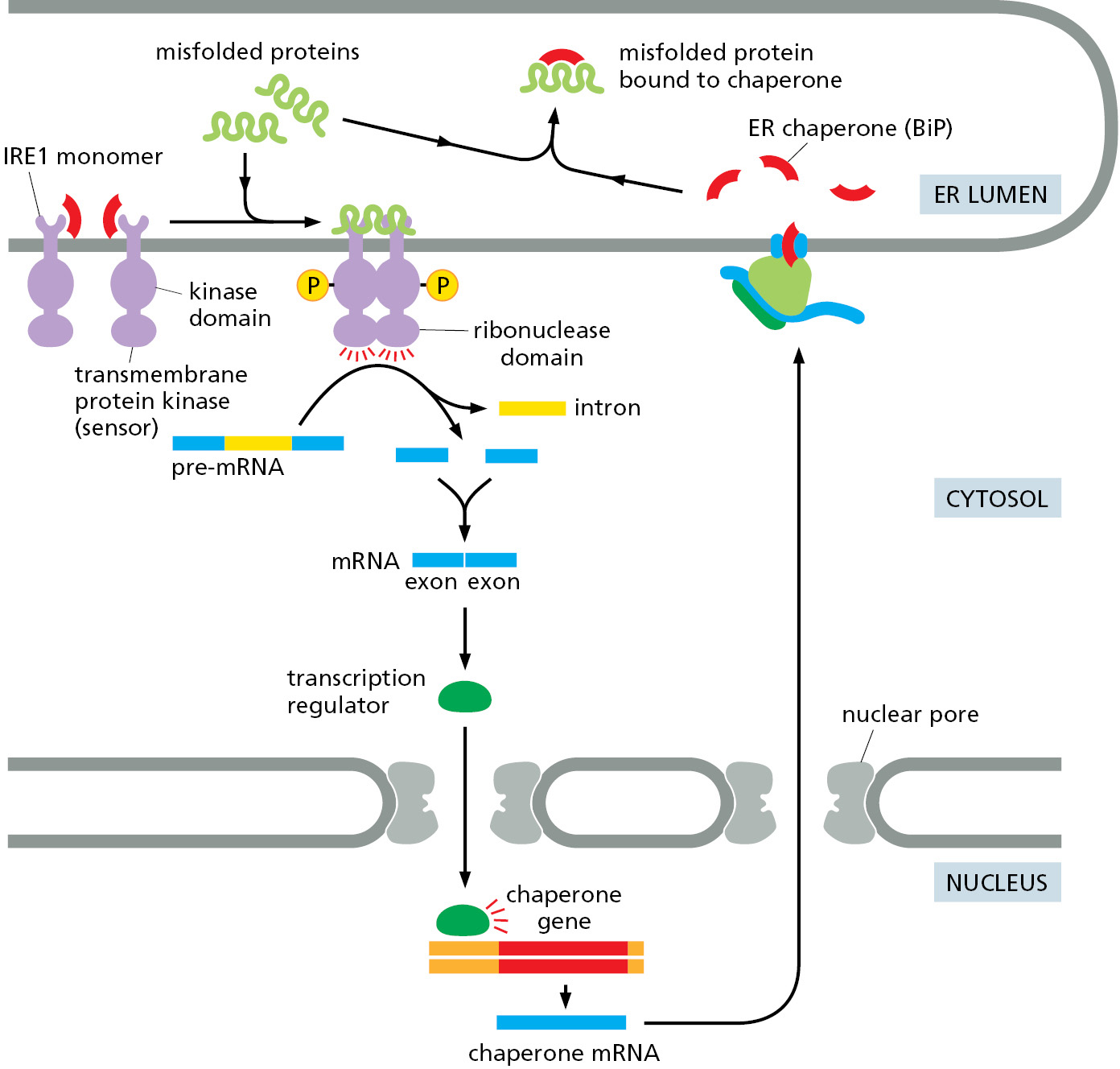
Misfolded proteins also activate a second transmembrane kinase in the ER, PERK. The target of activated PERK is a translation initiation protein whose phosphorylation has two consequences. First, translation of new proteins is reduced throughout the cell, thereby reducing the load of proteins that need to be folded in the ER. Second, some proteins are preferentially translated when translation initiation factors are scarce, and one of these is a transcription regulator that helps activate the transcription of the genes that execute the unfolded protein response.
Finally, a third transcription regulator, ATF6, is initially synthesized as a transmembrane ER protein. Because it is embedded in the ER membrane, it cannot activate the transcription of genes in the nucleus. When misfolded proteins accumulate in the ER, the ATF6 protein is transported to the Golgi apparatus. Resident proteases in the Golgi apparatus membrane cleave off the cytosolic domain of ATF6, which can now migrate to the nucleus and help activate the transcription of genes encoding proteins involved in the unfolded protein response. This mechanism of activation of a latent membrane-embedded transcription factor is similar to how the transcription regulator that controls cholesterol biosynthesis is activated (discussed later in this chapter). The relative importance of each of these three pathways in the unfolded protein response differs in different cell types, enabling each cell type to tailor the unfolded protein response to its particular needs.
The signaling pathways that execute the unfolded protein response are used during normal physiological conditions to adjust ER capacity to closely match demand for the ER. For example, insulin production increases substantially in pancreatic β cells in response to eating a meal. The elevated demand for the processing capacity of the ER, where insulin is initially assembled, partially activates PERK so cells can adjust insulin synthesis rates to avoid overburdening the ER. In another example, IRE1 is activated when B cells begin differentiating into antibody-secreting plasma cells. IRE1 activation dramatically expands the ER content of the cell in preparation for the very high levels of immunoglobulins that will soon be assembled there.
The unfolded protein response ultimately increases the production of proteins that improve protein processing in the ER and reduce the burden of misfolded proteins. As homeostasis is restored, the activities of IRE1, PERK, and ATF6 abate. If homeostasis cannot be restored, persistently active signaling from the ER, particularly via PERK, activates genes that initiate apoptosis. In multicellular organisms, it is often less detrimental to eliminate a persistently dysfunctional cell than risk its aberrant interactions with neighboring cells.
The ER Assembles Most Lipid Bilayers
The ER membrane is the site of synthesis of nearly all of the cell’s major classes of lipids, including both phospholipids and cholesterol, required for the production of new cell membranes. The major phospholipid made is phosphatidylcholine, which can be formed in three steps from choline, two fatty acids, and glycerol phosphate (Figure 12–38). Each step is catalyzed by enzymes in the ER membrane, which have their active sites facing the cytosol, where all of the required metabolites are found. Thus, phospholipid synthesis occurs exclusively in the cytosolic leaflet of the ER membrane. Because fatty acids are not soluble in water, they are shepherded from their sites of synthesis in the cytosol to the ER by a fatty acid binding protein. After arrival in the ER membrane and activation with CoA, acyl transferases successively add two fatty acids to glycerol phosphate to produce phosphatidic acid. Phosphatidic acid is sufficiently water-insoluble to remain in the lipid bilayer; it cannot be extracted from the bilayer by the fatty acid binding proteins. It is therefore this first step that enlarges the ER lipid bilayer. The later steps determine the head group of a newly formed lipid molecule and therefore the chemical nature of the bilayer, but they do not result in net membrane growth. The two other major membrane phospholipids—phosphatidylethanolamine and phosphatidylserine (see Figure 10–3)—as well as the minor phospholipid phosphatidylinositol (PI), are all synthesized in this way.
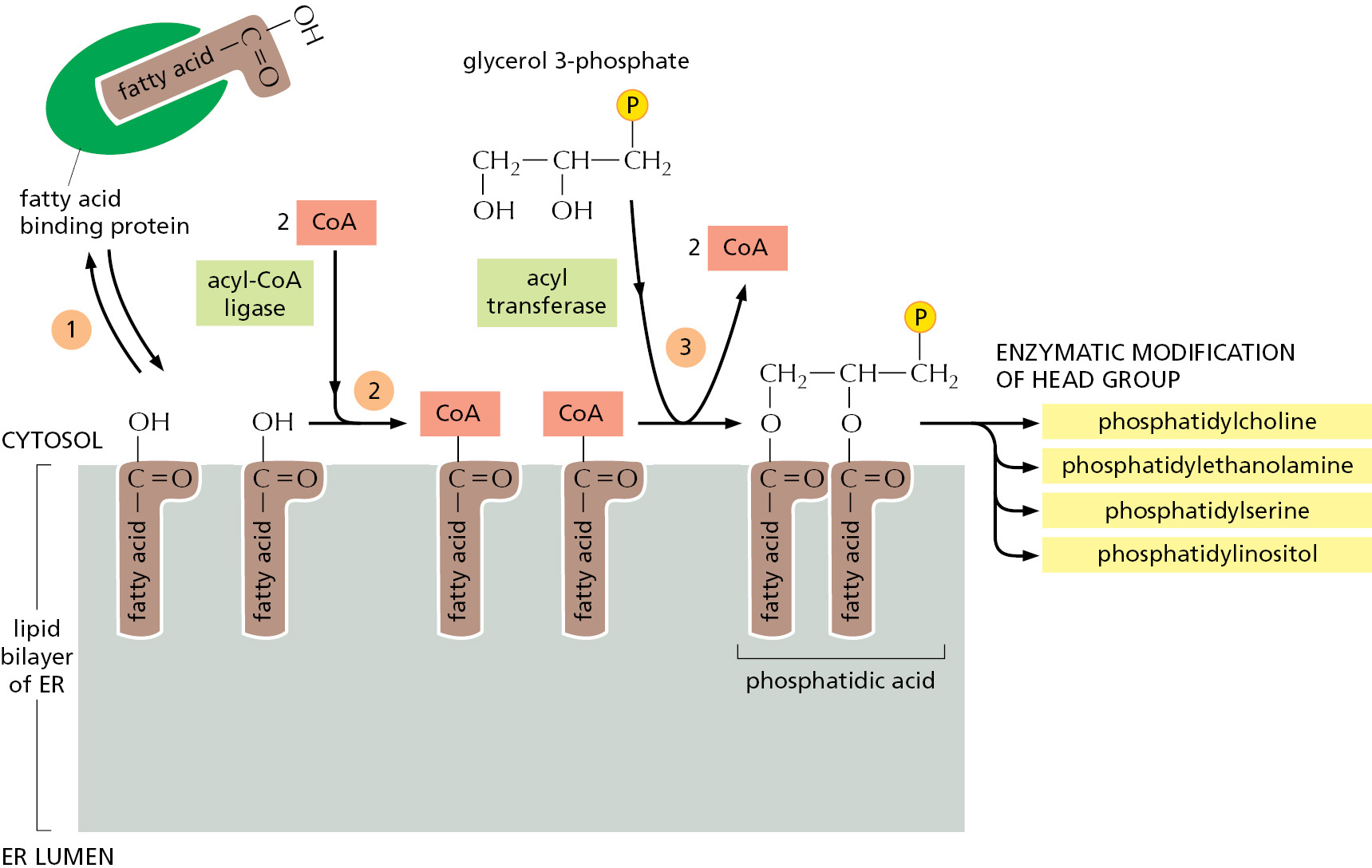
Because phospholipid synthesis takes place in the cytosolic leaflet of the ER lipid bilayer, there needs to be a mechanism that transfers some of the newly formed phospholipid molecules to the lumenal leaflet of the bilayer. In synthetic lipid bilayers, lipids do not “flip-flop” in this way (see Figure 10–10). In the ER, however, phospholipids equilibrate across the membrane within minutes, which is almost 100,000 times faster than can be accounted for by spontaneous “flip-flop.” This rapid trans-bilayer movement is mediated by a poorly characterized phospholipid translocator called a scramblase, which nonselectively equilibrates phospholipids between the two leaflets of the lipid bilayer (Figure 12–39). Thus, the different types of phospholipids are thought to be equally distributed between the two leaflets of the ER membrane.
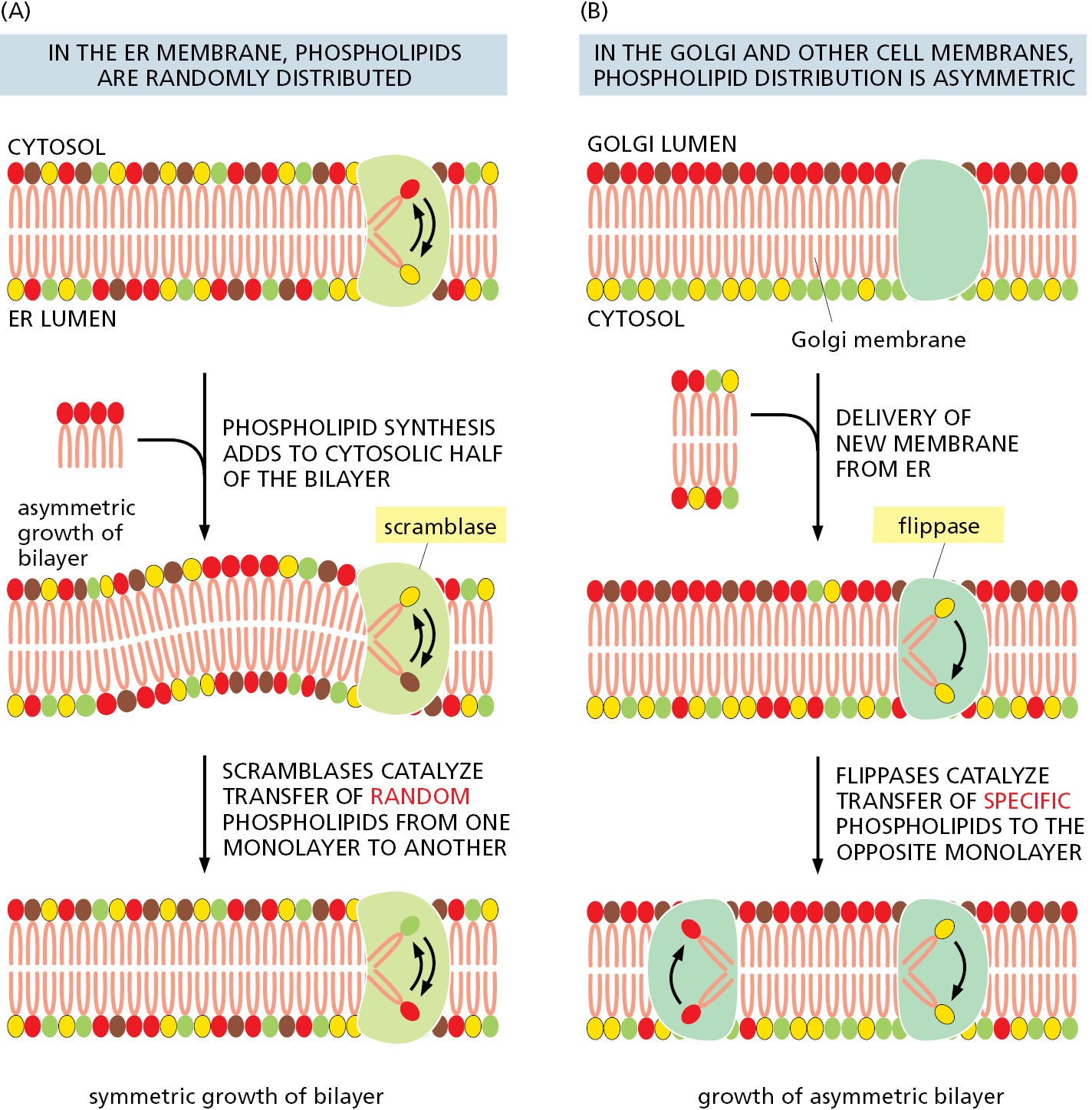
The ER also produces cholesterol and ceramide (Figure 12–40). Ceramide is made by condensing the amino acid serine with a fatty acid to form the amino alcohol sphingosine (see Figure 10–3); a second fatty acid is then covalently added to form ceramide. The ceramide is exported to the Golgi apparatus, where it serves as a precursor for the synthesis of two types of lipids. Glycosphingolipids (glycolipids; see Figure 10–16) are formed when oligosaccharides are added to ceramide, while sphingomyelin (discussed in Chapter 10) results from the addition of phosphocholine. Because glycolipids and sphingomyelin are both produced by enzymes that have their active sites exposed to the lumen of the Golgi apparatus, they are restricted to the noncytosolic leaflet of the lipid bilayers that contain them.
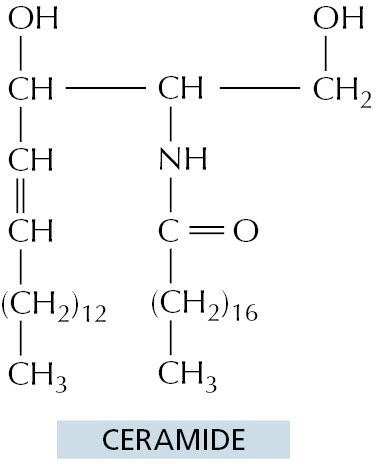
As discussed in Chapter 13, the plasma membrane and the membranes of the Golgi apparatus, lysosomes, and endosomes all form part of a membrane system that communicates with the ER by means of transport vesicles. A large proportion of the lipids that compose the membranes of these organelles is acquired via the membranes delivered by transport vesicles. Despite exchange of membrane lipids through vesicular transport, the lipid composition of each organellar membrane is distinct and contributes to its unique identity and functional properties. This specialization is achieved by a combination of three mechanisms. First, a transport vesicle can have a different lipid composition than the organelle it is departing, thereby delivering only a subset of lipids to its destination. Second, proteins in an organelle’s membrane can modify the head groups of certain lipids to change their identity (such as production of sphingomyelin from ceramide) or use flippases to move certain phospholipids from one leaflet of the membrane to the other (Figure 12–39B). Third, specific lipids can be selectively transferred from one membrane to another by nonvesicular transport routes as discussed next.
Membrane Contact Sites Between the ER and Other Organelles Facilitate Selective Lipid Transfer
Mitochondria and plastids do not communicate with the ER by vesicular transport, so they require different mechanisms to import many of their lipids from the ER for growth. Carrier proteins in the cytosol called lipid transfer proteins ferry individual lipid molecules between membranes, functioning much like fatty acid binding proteins that shepherd fatty acids through the cytosol (see Figure 12–38). In many cases, lipid transfer proteins function at organelle contact sites where the originating and destination membranes are held within ∼10–30 nm of each other by specific junction complexes. Different lipid transfer proteins shuttle phosphatidylcholine and phosphatidylserine from the ER to mitochondria at contact sites. Disruption of the junctional complexes or the lipid transfer proteins impairs lipid import into mitochondria and causes their dysfunction.
The extensive network of the ER participates in contact sites with most other cellular organelles (Figure 12–41). As with the ER–mitochondria contact sites (see Figure 12–16), one of the main functions of these other organelle contact sites is to exchange lipids (Figure 12–42). Cells contain several families of lipid transfer proteins. Each of these can typically bind one molecule of a specific lipid (or in some cases multiple related lipids) and has additional domains that can interact with specific cellular membranes. In this manner, they serve as shuttling proteins that have distinctive specificities for the donor and acceptor membranes and the lipid they transport. Contact sites between two organellar membranes favor recruitment of the lipid transfer protein that binds these membranes, thereby enhancing the efficiency of lipid exchange. Cholesterol uses a specialized transport system from lysosomes, where it is delivered as cholesterol esters in lipoproteins, to the plasma membrane and other locations in the cell (as we discuss in Chapter 13).
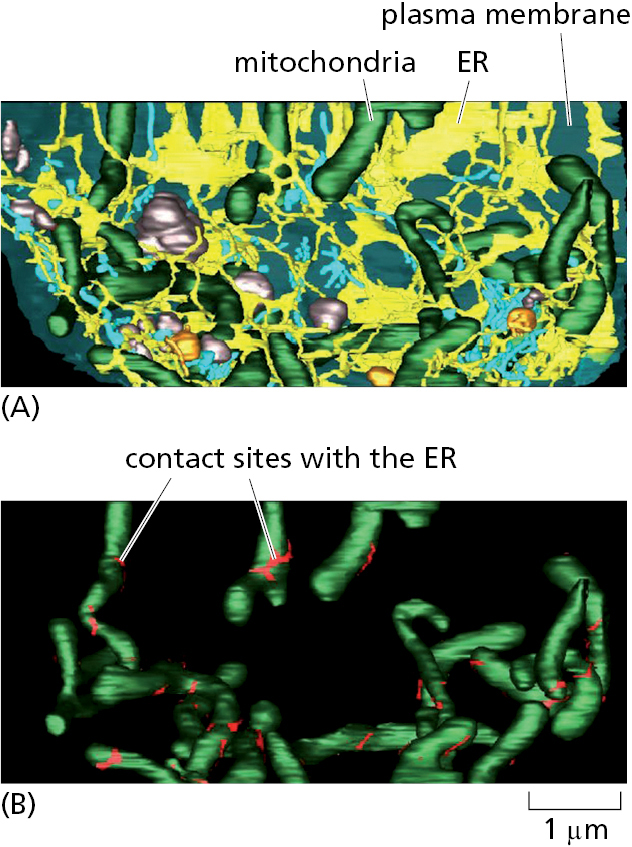
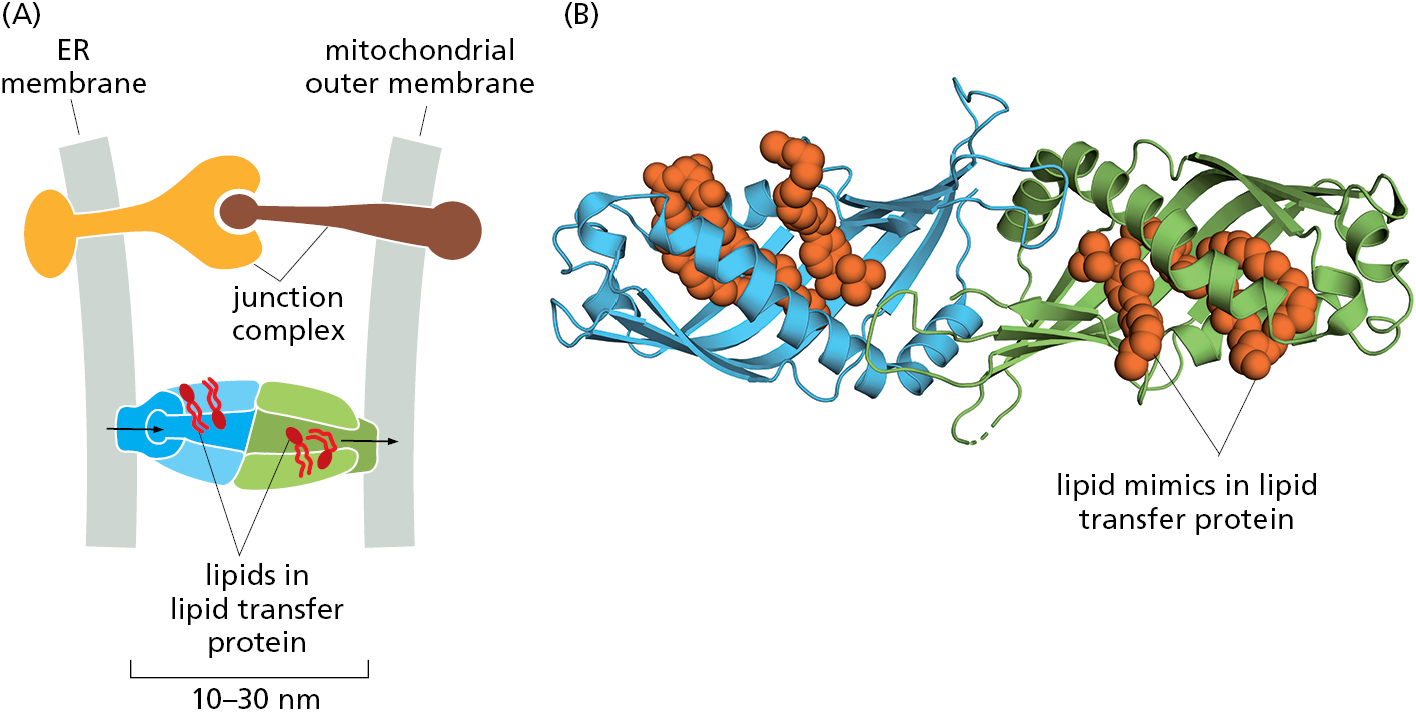
Summary
The extensive ER network serves as a factory for the production of almost all of the cell’s lipids. In addition, a major portion of the cell’s protein synthesis occurs on the cytosolic surface of the rough ER: virtually all proteins destined for secretion or for the ER itself, the Golgi apparatus, the lysosomes, the endosomes, and the plasma membrane are first imported into the ER from the cytosol. In the ER lumen, the proteins fold and oligomerize, disulfide bonds are formed, and N-linked oligosaccharides are added. The pattern of N-linked glycosylation is used to indicate the extent of protein folding, so that proteins leave the ER only when they are properly folded. Proteins that do not fold or oligomerize correctly are translocated back into the cytosol, where they are de-glycosylated, polyubiquitylated, and degraded in proteasomes. If misfolded proteins accumulate in excess in the ER, they trigger an unfolded protein response, which activates appropriate genes in the nucleus to help the ER cope.
Only proteins that carry a special ER signal sequence are imported into the ER. The signal sequence is recognized by a signal-recognition particle (SRP), which binds both the growing polypeptide chain and the ribosome and directs them to a receptor protein on the cytosolic surface of the rough ER membrane. This binding to the ER membrane initiates the translocation process that threads a loop of polypeptide chain across the ER membrane through the hydrophilic pore of a protein translocator.
Soluble proteins—destined for the ER lumen, for secretion, or for transfer to the lumen of other organelles—pass completely into the ER lumen. Transmembrane proteins destined for the ER or for other cell membranes become anchored in the ER membrane by one or more membrane-spanningα-helical segments in their polypeptide chains. As these hydrophobic portions of the protein emerge from the ribosome, they are recognized by the protein translocator, which provides a passageway into the membrane. When a polypeptide contains multiple hydrophobic segments, it will pass back and forth across the bilayer multiple times as a multipass transmembrane protein.
The asymmetry of protein insertion and glycosylation in the ER establishes the sidedness of the membranes of all the other organelles that the ER supplies with membrane proteins. Lipids are synthesized at the cytosolic face of the ER, equilibrate between both leaflets of the lipid bilayer, and are transported to other organelles often at interorganelle junctions by lipid transfer proteins localized there. Specific flippases establish and maintain lipid asymmetry in the plasma membrane, further contributing to its sidedness.
Glossary
- endoplasmic reticulum (ER) (ER)
- Extensive, net-like membrane-bounded compartment in the cytoplasm of eukaryotic cells, where lipids are synthesized and membrane-bound proteins and secretory proteins are made.
- ER lumen
- The space enclosed by the membrane of the endoplasmic reticulum (ER).
- rough ER (rough endoplasmic reticulum)
- Endoplasmic reticulum with ribosomes on its cytosolic surface. Involved in the synthesis of secreted and membrane-bound proteins.
- smooth ER (smooth endoplasmic reticulum)
- Region of the endoplasmic reticulum not associated with ribosomes. Involved in detoxification reactions, Ca
- organelle contact sites
- Region of contact between two organelles stabilized by specific tethering proteins.
- microsomes
- Small vesicle derived from endoplasmic reticulum that is produced by fragmentation when cells are homogenized.
- ER signal sequence
- N-terminal signal sequence that directs proteins to enter the endoplasmic reticulum (ER). Cleaved off by signal peptidase after entry.
- signal-recognition particle (SRP) (SRP)
- Ribonucleoprotein particle that binds an ER signal sequence on a partially synthesized polypeptide chain and directs the polypeptide and its attached ribosome to the endoplasmic reticulum.
- SRP receptor
- Ribonucleoprotein particle that binds an ER signal sequence on a partially synthesized polypeptide chain and directs the polypeptide and its attached ribosome to the endoplasmic reticulum.
- protein translocator
- Any membrane-bound protein that mediates the transport of another protein across a membrane.
- co-translational
- Occurring as translation proceeds. Examples include the import of a protein into the endoplasmic reticulum before the polypeptide chain is completely synthesized (co-translational translocation; Figure 12–24), and the folding of a nascent protein into its secondary and tertiary structure as it emerges from a ribosome (Figure 6–83C).
- Membrane-bound ribosomes
- Ribosome attached to the cytosolic face of the endoplasmic reticulum. The site of synthesis of proteins that enter the endoplasmic reticulum.
- Free ribosomes
- Ribosome that is free in the cytosol, unattached to any membrane.
- polyribosome
- mRNA engaged with multiple ribosomes in the act of translation.
- Sec61 complex
- Three-subunit core of the protein translocator that transfers polypeptide chains across the endoplasmic reticulum membrane.
- Cryo-electron microscopy
- Technique for examining a thin film of an aqueous suspension of biological material that has been frozen rapidly enough to create vitreous ice. The specimen is then kept frozen and transferred to the electron microscope. Image contrast is low, but this type of microscopy permits the determination of atomic-level structures because the image is generated solely by the macromolecular structures present.
- post-translational
- Occurring after completion of translation, thus after the completion of protein synthesis.
- transmembrane segments
- The region of a transmembrane protein that spans the lipid bilayer, often as an α helix.
- tail-anchored proteins
- Protein containing a transmembrane segment very close to its C-terminus.
- glycosylphosphatidylinositol anchor (GPI anchor)
- Lipid linkage by which some membrane proteins are bound to the membrane. The protein is joined, via an oligosaccharide linker, to a phosphatidylinositol anchor during its travel through the endoplasmic reticulum.
- ER resident proteins
- Protein that remains in the lumen of the endoplasmic reticulum (ER) or its membranes and carries out its function there, as opposed to the many proteins that are present in the ER only in transit.
- ER retention signal
- Short amino acid sequence on a protein that prevents it from moving out of the endoplasmic reticulum (ER). Found on those proteins that are resident in the ER and function there.
- BiP
- Endoplasmic reticulum (ER)-resident chaperone protein. Member in the family of hsp70-type chaperones.
- glycoproteins
- Any protein with one or more sugars or oligosaccharide chains covalently linked to amino acid side chains. Most secreted proteins and most proteins exposed on the outer surface of the plasma membrane are glycoproteins.
- protein glycosylation
- The process of transferring either a single saccharide or a preformed precursor oligosaccharide to proteins.
- dolichol
- Isoprenoid lipid molecule that anchors the precursor oligosaccharide in the endoplasmic reticulum membrane during protein glycosylation.
- oligosaccharyl transferase
- Endoplasmic reticulum (ER) enzyme complex that transfers core oligosaccharides from dolichol lipid anchors to selected asparagine side chains in newly synthesized proteins as they enter the ER lumen.
- calnexin
- Carbohydrate-binding chaperone protein in the endoplasmic reticulum (ER) membrane; binds to oligosaccharides on incompletely folded proteins and retains them in the ER.
- calreticulin
- Carbohydrate-binding chaperone protein in the endoplasmic reticulum (ER) lumen; binds to oligosaccharides on incompletely folded proteins and retains them in the ER.
- unfolded protein response
- The cellular response triggered by an accumulation of misfolded proteins in the endoplasmic reticulum. It causes an expansion of the ER and increased transcription of genes that code for endoplasmic reticulum chaperones and degradative enzymes, as well as other changes.