THE CHEMICAL COMPONENTS OF A CELL
Living organisms are made of only a small selection of the 92 naturally occurring elements, four of which—carbon (C), hydrogen (H), nitrogen (N), and oxygen (O)—make up 96.5% of an organism’s weight (Figure 2–1). The atoms of these elements are linked together by covalent bonds to form molecules (see Panel 2–1, pp. 94–95). Because covalent bonds are typically 100 times stronger than the thermal energies within a cell, they resist being pulled apart by thermal motions, and they are normally broken only during biologically catalyzed chemical reactions that are of use to the cell. Noncovalent bonds are much weaker (Figure 2–2), but sets of them allow molecules to recognize each other and reversibly associate, which is critical for the vast majority of biological functions.
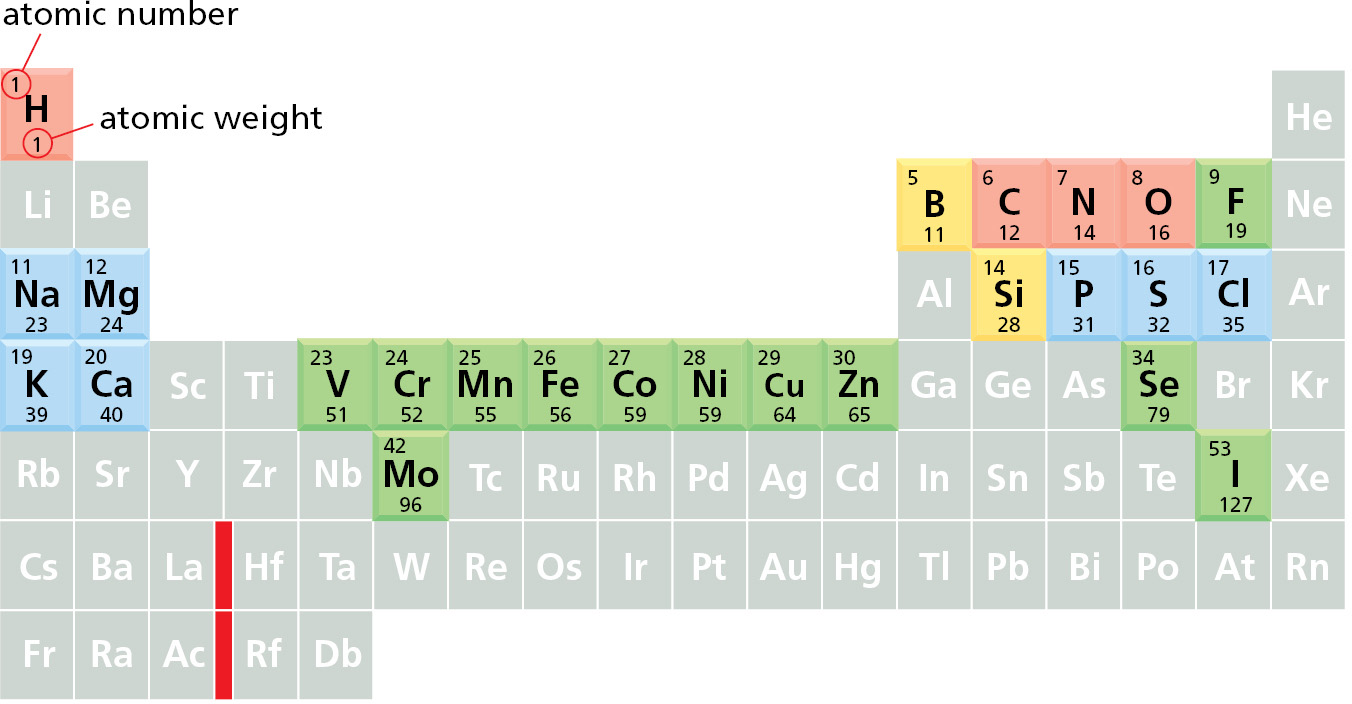
The four elements highlighted in red constitute 99% of the total number of atoms present in the human body (and 96.5% of its weight). An additional seven elements, highlighted in blue, together represent about 0.9% of the total atoms in our bodies. The elements shown in green are required in trace amounts by humans. It remains unclear whether those elements shown in yellow are essential in humans. The chemistry of life is therefore predominantly the chemistry of lighter elements. The atomic weights shown here are those of the most common isotope of each element. The vertical red line marks a break in the periodic table where a group of large atoms with similar chemical properties is omitted.
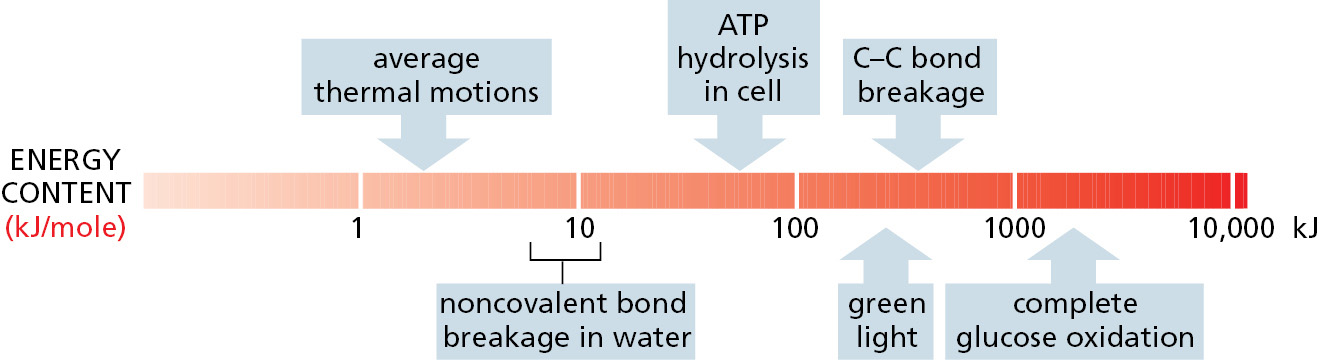
One joule (J) is the amount of energy required to move an object a distance of 1 meter (m) against a force of 1 newton (N). This measure of energy is derived from the SI units (Système International d’Unités) universally employed by physical scientists. A second unit of energy, often used by cell biologists, is the kilocalorie (kcal):1 calorie (cal) is the amount of energy needed to raise the temperature of 1 gram (g) of water by 1°C. One kilojoule (kJ) is equal to 0.239 kcal.
Water Is Held Together by Hydrogen Bonds
Because 70% of the weight of a cell is water, the reactions that make life possible occur in an aqueous environment. Life on Earth is thought to have begun in shallow bodies of water that had concentrated essential molecules, and the conditions in that primeval environment have left a permanent stamp on the chemistry of all living things.
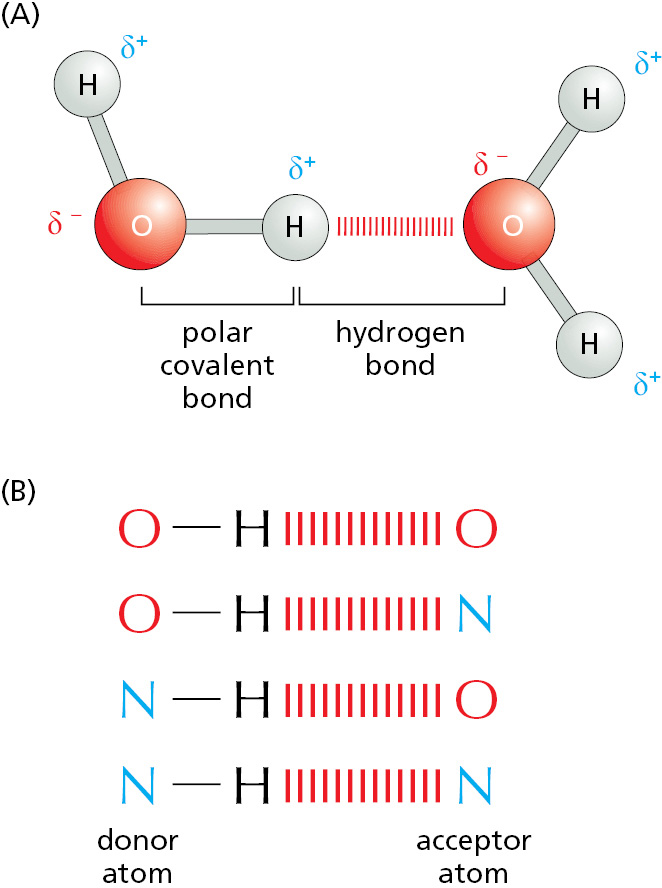
The chemical properties of water are reviewed in Panel 2–2 (pp. 96–97). In a water molecule (H2O), the two H atoms are linked to the O atom by covalent bonds that are highly polar, inasmuch as the O atom attracts electrons more strongly than does the H atom. Consequently, there is a preponderance of positive charge on the two H atoms and of negative charge on the O atom. When a positively charged region of one water molecule (that is, one of its H atoms) approaches a negatively charged region (that is, the O atom) of a second water molecule, the electrical attraction between them can result in a hydrogen bond (Figure 2–3A). These bonds are much weaker than covalent bonds and are easily broken by the random thermal motions that reflect the heat energy of the molecules. Thus, each bond lasts only a very short time. But the combined effect of many weak bonds can be profound. For example, each water molecule can form hydrogen bonds through its two H atoms to two other water molecules, producing a network in which hydrogen bonds are being continually broken and formed. It is only because of these hydrogen bonds that link water molecules together that water is a liquid at room temperature—with a high boiling point and high surface tension—rather than a gas.
Hydrogen bonds are not limited to water, and they are central to much of biology. This bond represents a special form of polar interaction in which an electropositive hydrogen atom is shared by two electronegative atoms. The hydrogen in this bond can be viewed as a proton that has partially dissociated from a donor atom, allowing it to be shared by a second, acceptor atom. Unlike a typical electrostatic interaction, this bond is highly directional—being strongest when a straight line can be drawn between all three of the involved atoms (Figure 2–3B).
Molecules, such as alcohols, that contain polar bonds and that can form hydrogen bonds with water dissolve readily in water. Molecules carrying charges (ions) likewise interact favorably with water. Such molecules are termed hydrophilic, meaning that they are water-loving. Many of the molecules in the aqueous environment of a cell necessarily fall into this category, including sugars, DNA, RNA, and most proteins. Hydrophobic (water-fearing) molecules, by contrast, are uncharged and form few or no hydrogen bonds, and so do not dissolve in water. Hydrocarbons are an important example. In these molecules, all of the H atoms are covalently linked to C atoms by a largely nonpolar bond; thus, they cannot form effective hydrogen bonds to other molecules (see Panel 2–1, pp. 94–95). This makes the hydrocarbon as a whole hydrophobic—a property that is exploited in cells, whose membranes are constructed from molecules that have long hydrocarbon tails, as we shall see in Chapter 10.
Four Types of Noncovalent Attractions Help Bring Molecules Together in Cells
Much of biology depends on the specific binding between different molecules caused by three types of noncovalent bonds—hydrogen bonds, electrostatic attractions (ionic bonds), and van der Waals attractions—combined with a fourth factor that can push molecules together: the hydrophobic force.
Electrostatic attractions are strongest when the atoms involved are fully charged, or ionized. But a weaker electrostatic attraction occurs between molecules that contain polar covalent bonds. Like hydrogen bonds, electrostatic attractions are extremely important in biology. For example, any large molecule with many polar groups will have a pattern of partial positive and negative charges on its surface. When such a molecule encounters a second molecule with a complementary set of charges, the two will be drawn to each other by electrostatic attraction.
In addition to hydrogen bonds and electrostatic attractions, a third type of noncovalent bond, called a van der Waals attraction, comes into play when any two atoms approach each other closely. These weak, nonspecific interactions are due to fluctuations in the distribution of electrons in every atom, which can generate a transient attraction when the atoms are in very close proximity. These attractions occur in all types of molecules, even those that are nonpolar.
The relative lengths and strengths of these three types of noncovalent bonds are compared to the length and strength of covalent bonds in Table 2–1, both in the presence and in the absence of water. Note that, because water forms competing interactions with the involved molecules, the strength of both electrostatic attractions and hydrogen bonds is greatly weakened inside of the cell.
TABLE 2–1 Covalent and Noncovalent Chemical Bonds |
||||
Strength (kJ/mole**) |
||||
Bond type |
Length (nm) |
In vacuum |
In water |
|
Covalent |
0.10 |
377 (90) |
377 (90) |
|
Noncovalent |
Ionic* |
0.25 |
335 (80) |
12.6 (3) |
Hydrogen |
0.17 |
16.7 (4) |
4.2 (1) |
|
van der Waals attraction (per atom) |
0.35 |
0.4 (0.1) |
0.4 (0.1) |
The fourth effect that often brings molecules together in water is not, strictly speaking, a bond at all. However, a very important hydrophobic force is caused by a pushing of nonpolar surfaces out of the hydrogen-bonded water network, where they would otherwise physically interfere with the highly favorable interactions between water molecules. Bringing any two nonpolar surfaces together reduces their contact with water, and in this sense, the force is nonspecific. Nevertheless, we shall see in Chapter 3 that hydrophobic forces are central to the proper folding of protein molecules.
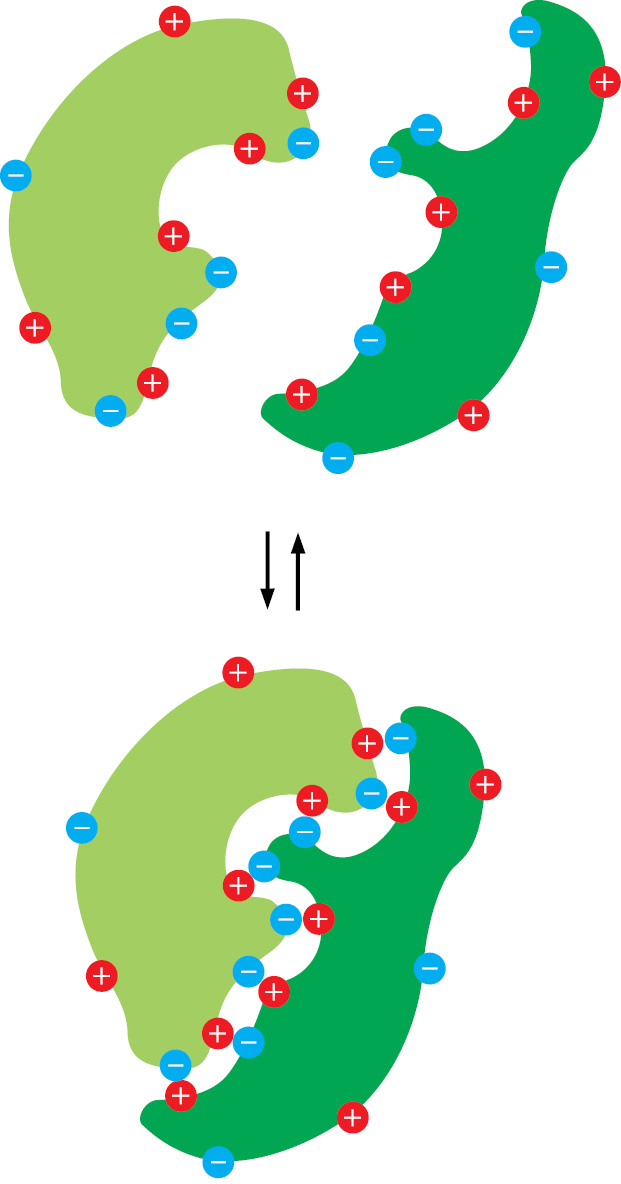
The properties of the four types of noncovalent attractions are presented in Panel 2–3 (pp. 98–99). Although each individual noncovalent attraction would be much too weak to be effective in the face of thermal motions, the energies of these noncovalent attractions can sum to create a strong force between two separate molecules. Thus, it is an entire set of noncovalent attractions that enables the complementary surfaces of two macromolecules to hold the two macromolecules together (Figure 2–4).
Some Polar Molecules Form Acids and Bases in Water
One of the simplest kinds of chemical reaction, and one that has a considerable significance for cells, takes place when a molecule containing a highly polar covalent bond between a hydrogen and another atom dissolves in water. The hydrogen atom in such a molecule has given up its electron almost entirely to the companion atom, and so exists as an almost naked positively charged hydrogen nucleus; in other words, a proton (H+). When this polar molecule becomes surrounded by water molecules, the proton will be attracted to the partial negative charge on the O atom of an adjacent water molecule. The proton can easily dissociate from its original partner and associate instead with the oxygen atom of the water molecule, generating a hydronium ion (H3O+) (Figure 2–5A). The reverse reaction also takes place very readily, so in an aqueous solution protons are constantly flitting to and fro between one molecule and another.
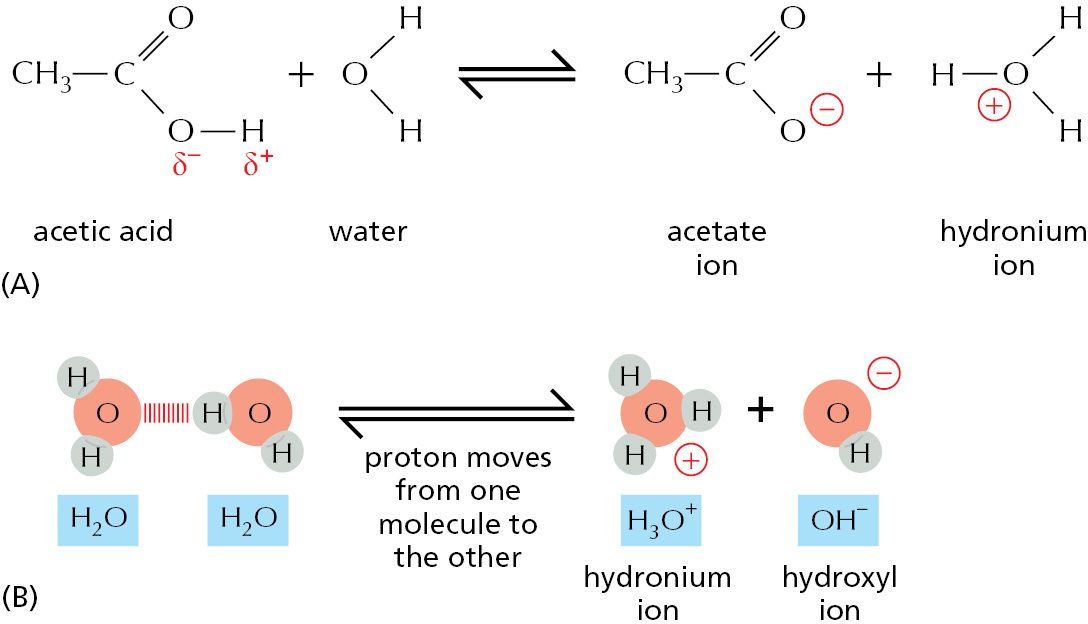
Substances that release protons when they dissolve in water, thus forming H3O+, are termed acids. The higher the concentration of H3O+, the more acidic the solution. H3O+ is present even in pure water, at a concentration of 10–7 M, as a result of the movement of protons from one water molecule to another (Figure 2–5B). By convention, the H3O+ concentration is usually referred to as the H+ concentration, even though most protons in an aqueous solution are present as H3O+. As explained in Panel 2–2, to avoid the use of unwieldy numbers the concentration of H3O+ is expressed using a logarithmic scale called the pH scale. Pure water has a pH of 7.0 and is said to be neutral; that is, neither acidic (pH < 7) nor basic (pH > 7).
Acids are characterized as being strong or weak, depending on how readily they give up their protons to water. Strong acids, such as hydrochloric acid (HCl), easily lose their protons. Acetic acid, on the other hand, is a weak acid because it holds on to its proton more tightly when dissolved in water. Many of the acids important in the cell—such as molecules containing a carboxyl (COOH) group—are weak acids.
Because the proton of a hydronium ion can be passed readily to many types of molecules in cells, altering their character, the concentration of H3O+ inside a cell (the acidity) must be closely regulated. Acids—especially weak acids—will give up their protons more readily if the concentration of H3O+ in solution is low and will tend to receive them back if the concentration in solution is high.
The opposite of an acid is a base. Any molecule capable of accepting a proton from a water molecule is called a base. Sodium hydroxide (NaOH) is basic (the term alkaline is also used) because it dissociates readily in aqueous solution to form Na+ ions and OH– ions. Because of this property, NaOH is called a strong base. More important in living cells, however, are the weak bases—those that have a weak tendency to reversibly accept a proton from water. Many biologically important molecules contain an amino (NH2) group. This group is a weak base that can generate OH– by taking a proton from water: –NH2 + H2O → –NH3+ + OH– (see Panel 2–2, pp. 96–97).
Because an OH– ion combines with an H3O+ ion to form two water molecules, any increase in the OH– concentration forces a decrease in the concentration of H3O+, and vice versa. Thus the product of the two values, [OH–] × [H3O+], is always 10–14 (moles/liter)2. A pure solution of water contains an equal concentration (10–7 M) of both ions, rendering it neutral. The interior of a cell is also kept close to neutrality by the presence of buffers: weak acids and bases that can release or take up protons near pH 7, keeping the environment of the cell relatively constant under a variety of conditions.
A Cell Is Formed from Carbon Compounds
Having briefly reviewed the ways that atoms combine into molecules and how these molecules behave in an aqueous environment, we now examine the main classes of small molecules found in cells. We shall see that a few categories of molecules, formed from a handful of different elements, give rise to all the extraordinary richness of form and behavior shown by living things.
If we disregard water and inorganic ions such as potassium, nearly all the molecules in a cell are based on carbon. Carbon is outstanding among all the elements in its ability to form large molecules; silicon is a poor second. Because carbon is small and has four electrons and four vacancies in its outermost shell, a carbon atom can form four covalent bonds with other atoms. Most important, one carbon atom can join to other carbon atoms through highly stable covalent C–C bonds to form chains and rings and hence generate large and complex molecules with no obvious upper limit to their size. The carbon compounds made by cells are called organic molecules. In contrast, all other molecules, including water, are said to be inorganic.
Certain combinations of atoms, such as the methyl (–CH3), hydroxyl (–OH), carboxyl (–COOH), carbonyl (–C═O), phosphate (–PO32–), sulfhydryl (–SH), and amino (–NH2) groups, occur repeatedly in the molecules made by cells. Each such chemical group has distinct chemical and physical properties that influence the behavior of the molecule in which the group occurs. The most common chemical groups and some of their properties are summarized in Panel 2–1 (pp. 94–95).
Cells Contain Four Major Families of Small Organic Molecules
The small organic molecules of the cell are carbon-based compounds that have masses in the range of 100–1000 daltons and contain up to 30 or so carbon atoms. They are usually found free in solution and have many different fates. Some are used as monomer subunits to construct the giant polymeric macromolecules that make up most of the mass of the cell—proteins, nucleic acids, and large polysaccharides. Others act as energy sources and are broken down and transformed into other small molecules in a maze of intracellular metabolic pathways. Many small molecules have more than one role in the cell; for example, acting both as a potential subunit for a macromolecule and as an energy source. Small organic molecules account for only about one-tenth of the total mass of organic matter in a cell, but they are very diverse. Nearly 4000 different kinds of small organic molecules have been detected in the well-studied bacterium, Escherichia coli.
All organic molecules are synthesized from and are broken down into the same set of simple compounds. As a consequence, the compounds in a cell are chemically related and most can be classified into a few distinct families. Broadly speaking, cells contain four major families of small organic molecules: the sugars, the fatty acids, the nucleotides, and the amino acids (Figure 2–6). Although many compounds present in cells do not fit into these categories, these four families of small organic molecules, together with the macromolecules made by linking them into long chains, account for a large fraction of the cell mass.
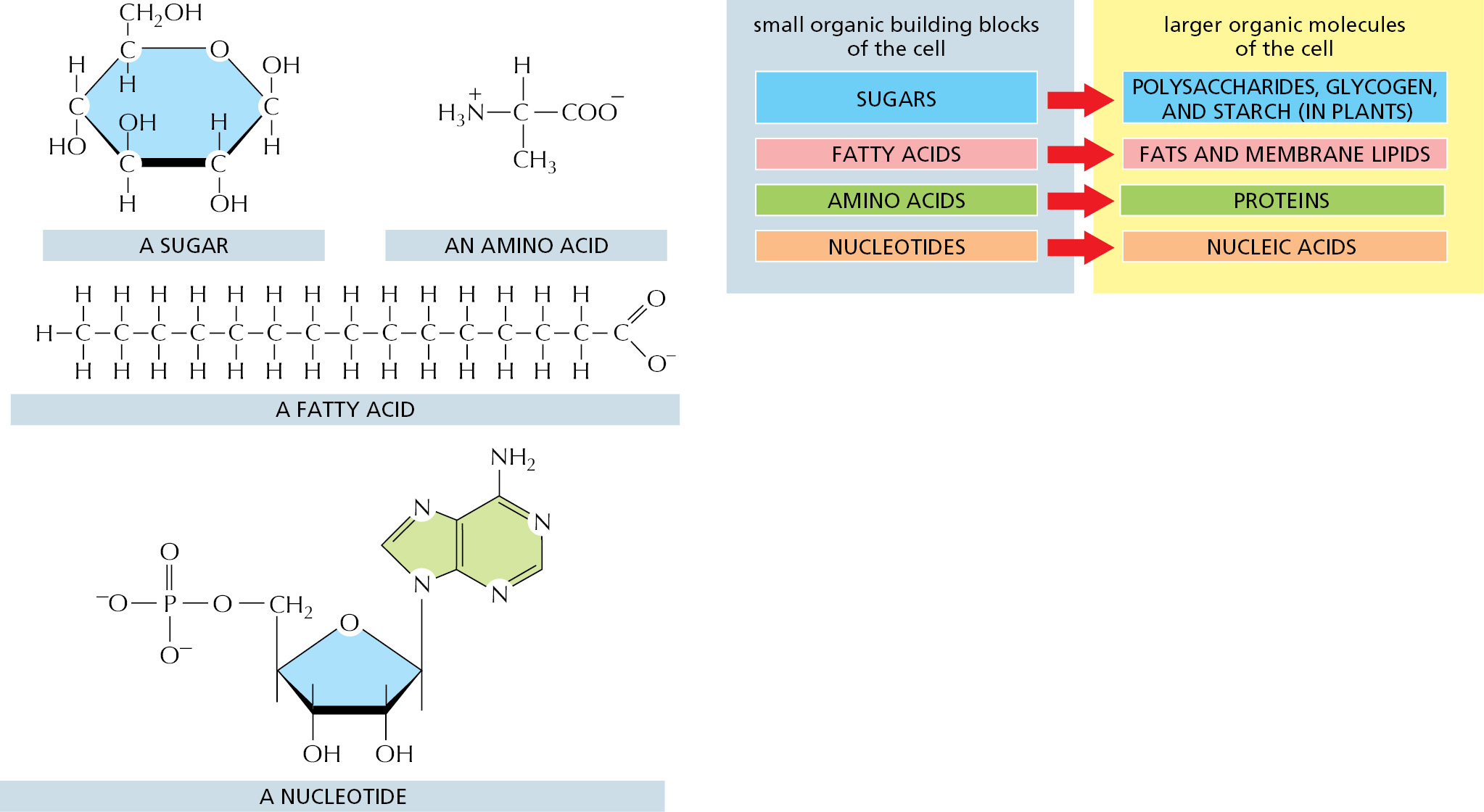
Amino acids and the proteins that they form will be the subject of Chapter 3. A summary of the structures and properties of the remaining three families—sugars, fatty acids, and nucleotides—is presented in Panels 2–4, 2–5, and 2–6, respectively (see pp. 100–105).
The Chemistry of Cells Is Dominated by Macromolecules with Remarkable Properties
By weight, macromolecules are the most abundant carbon-containing molecules in a living cell (Figure 2–7). They are the principal components from which a cell is constructed, and they also determine the most distinctive properties of living organisms. The macromolecules in cells are polymers that are constructed by covalently linking small organic molecules (called monomers) into long chains (Figure 2–8). They have remarkable properties that could not have been predicted from their simple constituents.
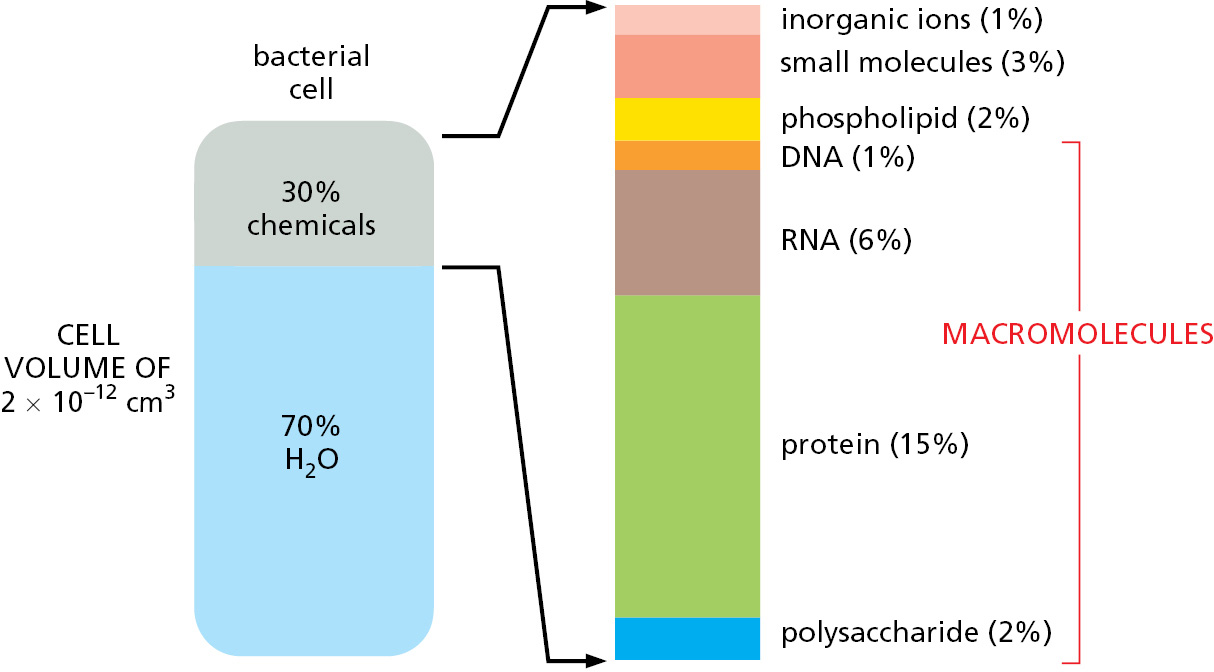
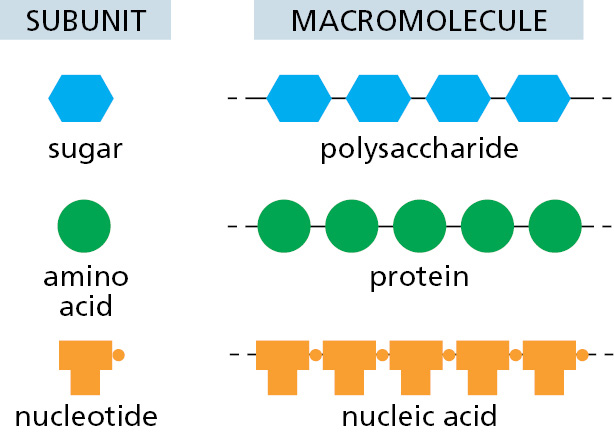
Proteins are abundant and spectacularly versatile, performing thousands of distinct functions in cells. Many proteins serve as enzymes, the catalysts that facilitate the many covalent bond-making and bond-breaking reactions that the cell needs. Enzymes catalyze all of the reactions in which cells extract energy from food molecules, for example. Other proteins are used to build structural components, such as tubulin, a protein that self-assembles to make the cell’s long microtubules, or histones, proteins that compact the DNA in chromosomes. Many proteins serve as signaling devices, producing networks that control cell functions. Yet other proteins act as molecular motors to produce force and movement, as for myosin in muscle. We shall describe the remarkable chemistry that underlies these diverse roles throughout this book.
Although the chemical reactions that add subunits to each polymer are different in detail for proteins, nucleic acids, and polysaccharides, they share important features. Each polymer grows by the addition of a monomer onto the end of a growing chain in a condensation reaction, in which one molecule of water is lost with each subunit added (Figure 2–9). The stepwise polymerization of monomers into a long chain is a simple way to manufacture a large, complex molecule, because the subunits are added by the same reaction performed over and over again by the same set of enzymes. Apart from some of the polysaccharides, most macromolecules are made from a limited set of monomers that are slightly different from one another; for example, the 20 different amino acids from which proteins are made. It is critical to life that the polymer chain is not assembled at random from these subunits; instead, the subunits are added in a precise order, or sequence. The elaborate mechanisms that allow enzymes to accomplish this task are described in detail in Chapters 5 and 6.
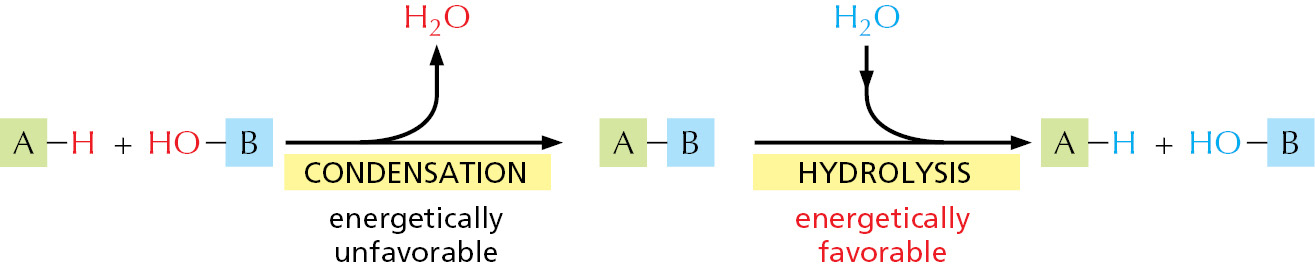
Noncovalent Bonds Specify Both the Precise Shape of a Macromolecule and Its Binding to Other Molecules
Most of the covalent bonds in a macromolecule allow rotation of the atoms they join, giving the polymer chain great flexibility. In principle, this allows a macromolecule to adopt an almost unlimited number of shapes, or conformations, as random thermal energy causes the polymer chain to writhe and rotate. However, the shapes of most biological macromolecules are highly constrained because of the many weak noncovalent bonds that form between different parts of the same molecule. If these noncovalent bonds are formed in sufficient numbers, the polymer chain can strongly prefer one particular conformation, determined by the linear sequence of monomers in its chain. Most protein molecules and many of the small RNA molecules found in cells fold tightly into a highly preferred conformation in this way (Figure 2–10).
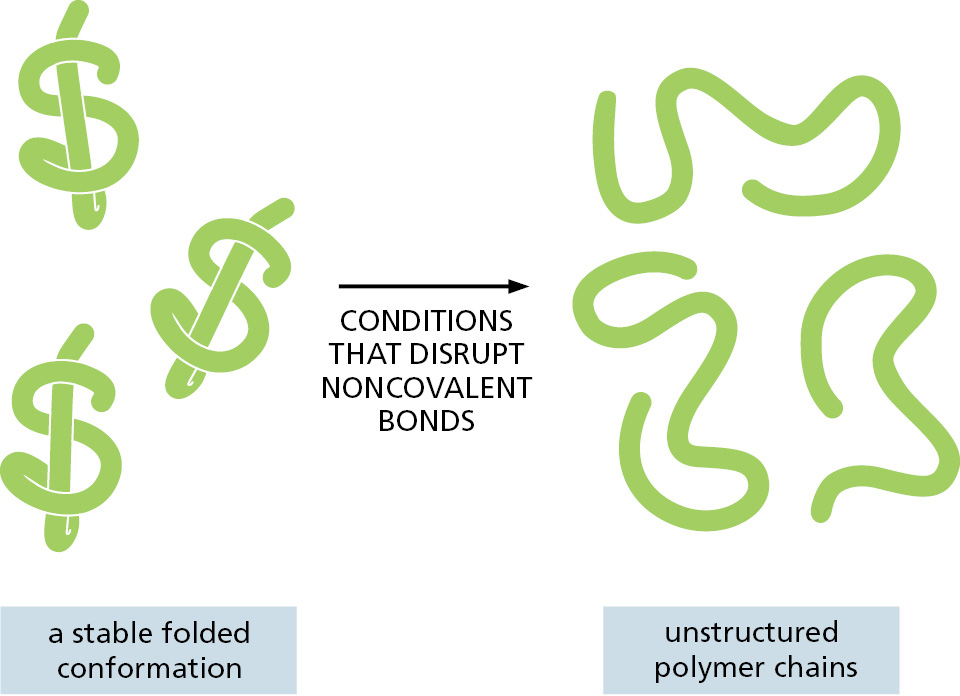
The four types of noncovalent interactions important in biological molecules were presented earlier (see also Panel 2–3, pp. 98–99). In addition to folding biological macromolecules into unique shapes, they can also add up to create a strong attraction between two different molecules (see Figure 2–4). This form of molecular interaction provides for great specificity, inasmuch as the close multipoint contacts required for strong binding make it possible for a macromolecule to select out—through binding—just one of the many thousands of types of molecules present inside a cell. Moreover, because the strength of the binding depends on the number of noncovalent bonds that are formed, interactions of almost any affinity are possible—allowing rapid dissociation where appropriate.
As we discuss next, binding of this type underlies all biological catalysis, making it possible for proteins to function as enzymes. In addition, noncovalent interactions allow macromolecules to be used to build larger structures, thereby forming intricate machines with multiple moving parts that perform such complex tasks as DNA replication and protein synthesis (Figure 2–11).
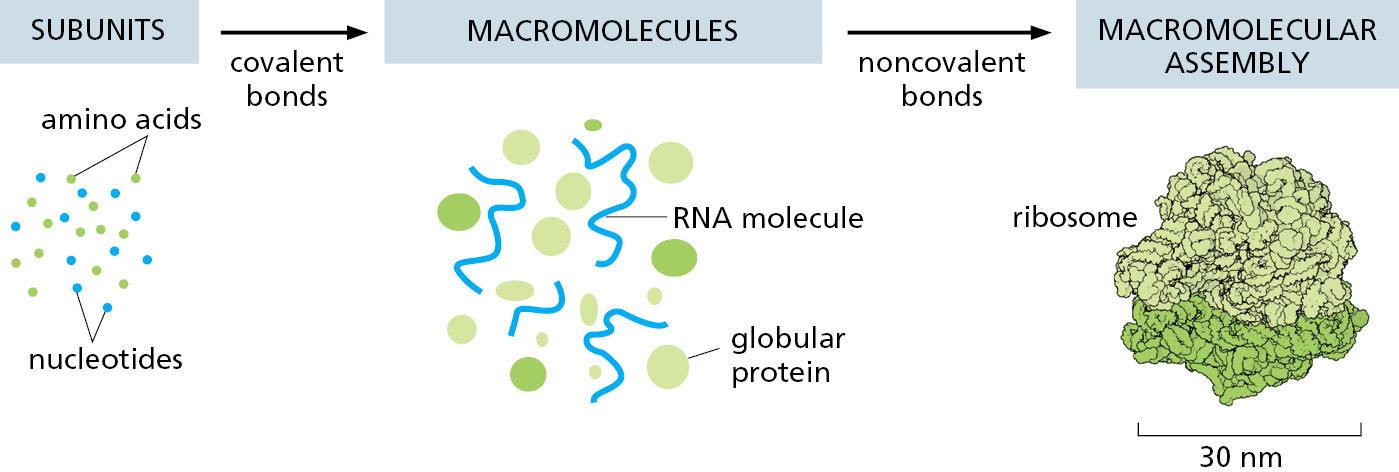
Summary
Living organisms are autonomous, self-propagating chemical systems. They are formed from a distinctive and restricted set of small carbon-based molecules that are essentially the same for every living species. Each of these small molecules is composed of a set of atoms linked to each other in a precise configuration through covalent bonds. The main categories are sugars, fatty acids, amino acids, and nucleotides.
Most of the dry mass of a cell consists of macromolecules that have been produced as linear polymers of amino acids (proteins) or nucleotides (DNA and RNA), covalently linked to each other in an exact order. Most of the protein molecules and many of the RNAs fold into a particular conformation that is determined by their sequence of subunits. This folding process creates unique surfaces, and it depends on a large set of weak attractions produced by noncovalent forces between atoms. These forces are of four types: electrostatic attractions, hydrogen bonds, van der Waals attractions, and an attraction between nonpolar groups caused by their hydrophobic expulsion from water. The same set of weak forces governs the specific binding of a macromolecule to both small molecules and other macromolecules, producing the myriad associations between biological molecules that generate the structure and the chemistry of a cell.
Glossary
- covalent bond
- Stable chemical link between two atoms produced by sharing one or more pairs of electrons.
- hydrogen bond
- Noncovalent bond in which an electropositive hydrogen atom is partially shared by two electronegative atoms.
- hydrophilic
- Dissolving readily in water. Literally, “water loving.”
- hydrophobic
- (lipophilic) Not dissolving readily in water. Literally, “water-fearing.”
- noncovalent bond
- Weak bonds that require multiple sets to hold two molecules together. Includes hydrogen bonds, electrostatic attractions, and van der Waals attractions.
- electrostatic attraction
- A noncovalent, ionic attraction between two molecules carrying groups of opposite charge.
- van der Waals attraction
- Type of (individually weak) noncovalent bond that is formed at close range between nonpolar atoms. (Table 2–1, p. 51, and Panel 2–3, pp. 98–99)
- hydrophobic force
- Force exerted by the hydrogen-bonded network of water molecules that brings two nonpolar surfaces together by excluding water between them.
- hydronium ion (H3O+)
- Water molecule associated with an additional proton. The form generally taken by protons in aqueous solution.
- proton (H+)
- Positively charged subatomic particle that forms part of an atomic nucleus. Hydrogen has a nucleus composed of a single proton (H+).
- acid
- A proton donor. Substance that releases protons (H+) when dissolved in water, forming hydronium ions (H3O+) and lowering the pH.
- pH scale
- Common measure of the acidity of a solution: “p” refers to power of 10, “H” to hydrogen. Defined as the negative logarithm of the hydrogen ion concentration in moles per liter (M). pH = –log[H+]. Thus a solution of pH 3 will contain 10–3 M hydrogen ions. pH less than 7 is acidic and pH greater than 7 is alkaline.
- base
- (1) A substance that can reduce the number of protons in solution, either by accepting H+ ions directly or by releasing OH– ions, which then combine with H+ to form H2O. (Chapter 2) (2) The purines and pyrimidines in DNA and RNA are organic nitrogenous bases and are commonly referred to simply as bases.
- chemical group
- Certain common combinations of atoms—such as methyl (-CH3), hydroxyl (-OH), carboxyl (-COOH), carbonyl (-C=O), phosphate (-PO32–), sulfhydryl (-SH), and amino (-NH2) groups—that have distinct chemical and physical properties and influence the behavior of the molecule in which the group occurs.
- macromolecule
- Polymers constructed of long chains of covalently linked, small organic (carbon-containing) molecules. The principal building blocks from which a cell is constructed and the components that confer the most distinctive properties of living things.
- buffer
- Solution of weak acid or weak base that resists the pH change that would otherwise occur when small quantities of acid or base are added.
Endnotes
- An ionic bond is an electrostatic attraction between two fully charged atoms. Return to reference *
- Values in parentheses are kcal/mole. 1 kJ = 0.239 kcal and 1 kcal = 4.18 kJ. Return to reference **