Microscopy: Optics and Properties of Light
2.1 Observing Microbes
How did people first see microbes? As we saw in Chapter 1, the microscope of Antonie van Leeuwenhoek first revealed the tiny life forms on his teeth; his superior lenses were key to his success. Since the time of Leeuwenhoek, microscopists have devised ever-more-powerful instruments to find microbes in familiar and unexpected habitats.
For example, an unusual spiral-shaped methane-oxidizing bacterium (methanotroph) was isolated from a peat bog in northern Russia (Fig. 2.1). Methane-oxidizing bacteria are crucial for curbing the release of methane from archaea that produce it. These bacteria were visualized as a whole by phase-contrast microscopy (Fig. 2.1A). Phase contrast makes use of the wave property of light, which causes light rays to bend “out of sync” at the edge between a microbe and its surrounding medium, thus generating high contrast. The patterns of light and dark reveal the cell’s spiral curves. Phase contrast is explained further in Section 2.3.
To peek inside a cell requires more powerful tools, such as electron microscopy (EM) (Fig. 2.1B). Transmission electron microscopy reveals the amazing pattern of membranes that contain the methane-oxidizing electron transport system. For transmission EM, the sample is sliced into very thin sections, stained with electron-dense metal atoms, and bombarded with a beam of electrons (see Section 2.6). Within the cell, the electron beam reveals surprising layers of membranes stacked like pancakes. These intracellular membranes are packed with proteins that transfer electrons from methane (CH4) onto oxygen (O2), a process discussed in Chapter 14. Ultimately much of the methane is trapped in the cell’s biomass, thus preventing escape of a greenhouse gas into the atmosphere.
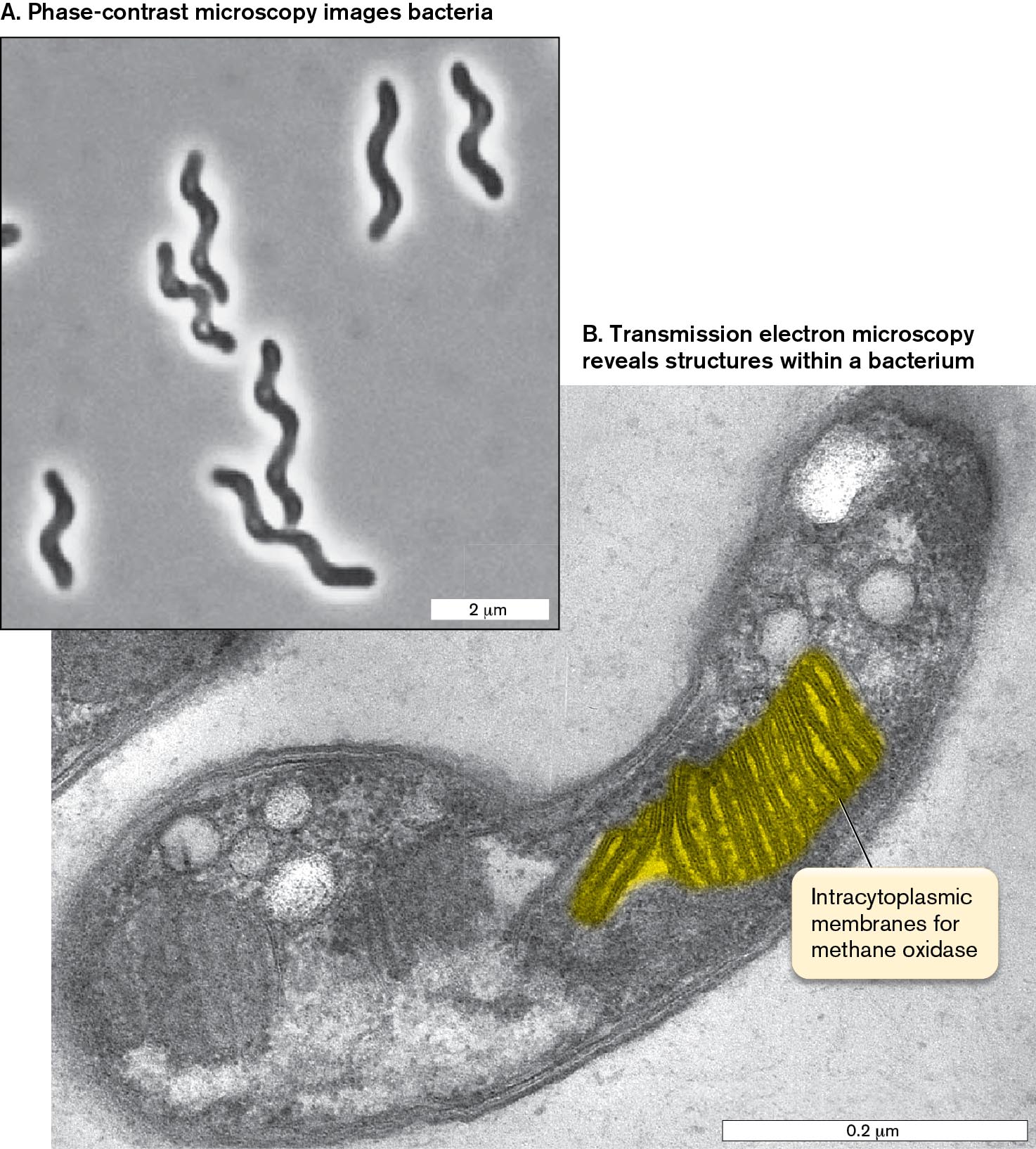
More information
Two micrographs of methane-oxidizing bacteria are shown, the first at low magnification and the second at high magnification.
The first micrograph shows several spiral shaped bacteria at low magnification. The bacteria are about 4 micrometers long and 0.25 micrometer wide.
The second micrograph shows a bacterium at high magnification with a methane oxidizing structure highlighted. The bacterium is 0.6 micrometer long and 0.1 micrometer wide. Internal structures are visible. A coiled structure is highlighted and described as the intracytoplasmic membranes for methane oxidase.
But why can’t we see microbes without magnification? The answer is surprisingly complex. In fact, our definition of “microscopic” is based on the properties of our eyes. We define what is visible and what is microscopic in terms of the human eye (Fig. 2.2).
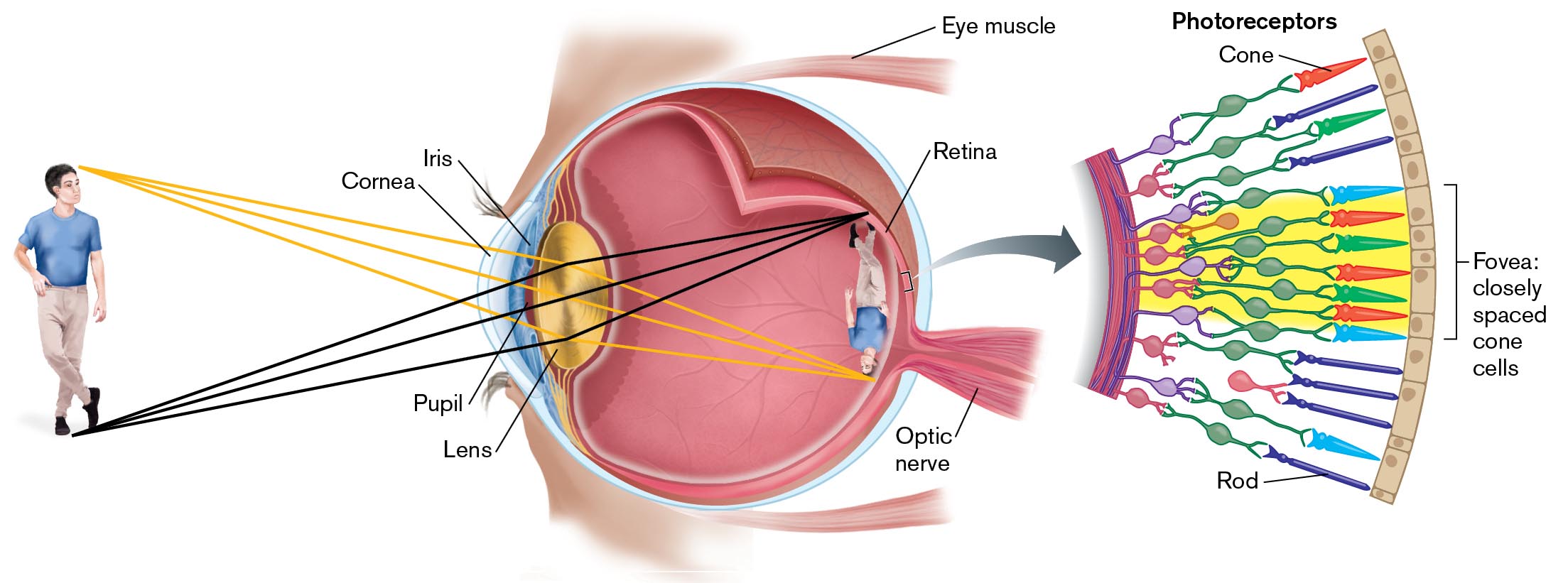
More information
An illustration depicts the principles of human vision. A clear outer layer, the cornea, surrounds the eye. The iris is the colored part of the eye and is slightly more internal than the cornea. The area between cornea and iris is the pupil. More internally is the lens. Along the back lining of the eye is the retina. A tail-like structure at the back of the eye is labeled as the optic nerve, and two fin shaped structures at the sides of the eye are labeled as eye muscle.
A man is standing in front of the eye. A smaller image of the man is mirrored and upside down on the retina. An arrow points toward an illustration describing the retina in detail. The illustration is labeled photoreceptors. Several cones and rod-shaped images are connected between the fovea, a section of closely spaced cone cells.
Resolution of Objects by Our Eyes
What determines the smallest object we can see? The size at which objects become visible depends on the eye’s ability to resolve detail. Resolution is the smallest distance between two objects that allows us to see them as separate objects. The eyes of humans and other animals observe an object by focusing its image on a retina packed with light-absorbing photoreceptor cells (Fig. 2.2 ). The image appears sharp, in focus, if the eye’s lens and cornea bend all the light rays from each point of the object to converge at one point on the retina. Nearby points are then resolved as separate.
In the human eye, the finest resolution of two separate points is perceived by the fovea, the portion of the retina where the photoreceptors are packed at the highest density. The foveal photoreceptors are cone cells, which detect primary colors (red, green, or blue) and finely resolved detail. A group of cones with their linked neurons forms one unit of detection, comparable to a pixel on a computer screen. The distance between two foveal “pixels” (groups of cones with neurons) limits our resolution to 100–200 micrometers (µm); that is, one- or two-tenths of a millimeter. So, a tenth of a millimeter is about the smallest object that most of us can see (resolve distinctly) without a magnifier.
What if our eyes were formed differently? The retinas of eagles have cones packed more closely than ours, so an eagle can resolve objects eight times as small (or eight times as far away) as a human can; hence, the phrase “eagle-eyed” means “sharp-sighted.” On the other hand, insect compound eyes have photoreceptors farther apart than ours, so insect eyes have poorer resolution. The best they can do is resolve objects 100-fold larger than those we can resolve. If a science-fictional giant ameba had eyes with photoreceptors 2 meters apart, it would perceive humans as “microscopic.”
Thought Question
2.1 As shown in Figure 2.2, the image passing through your cornea and lens is inverted (rotated upside down) on your retina. Why, then, does the world appear right side up?
ANSWER
ANSWER
The human brain interprets the image from the retina. Based on this interpretation, the brain knows that the image is upside down and inverts it to appear right side up. Researchers have tested what happens if an experimental subject wears special glasses that invert the image before the retina. At first, the brain sees everything upside down. After several days, the brain inverts the image perceived through the glasses, so it appears right side up. When the glasses are removed, the brain again takes time to restore its perception to right side up.
Note: In this book, we use standard metric units for size:
1 millimeter (mm) = one-thousandth of a meter (m) = 10−3 m
1 micrometer (µm) = one-thousandth of a millimeter = 10−6 m
1 nanometer (nm) = one-thousandth of a micrometer = 10−9 m
1 picometer (pm) = one-thousandth of a nanometer = 10−12 m
Some authors still use the traditional unit angstrom (Å), which equals a tenth of a nanometer, or 10−10 meter.
Resolution Differs from Detection
Can we detect the presence of objects whose size we cannot resolve? Yes, we can detect their presence as a group. For example, our eyes can detect a large population of microbes, such as a spot of mold on a piece of bread (about a million cells) or a cloudy tube of bacteria in liquid culture (a hundred million cells per milliliter; Fig. 2.3A). Detection, the ability to determine the presence of an object, differs from resolution. When the unaided eye detects the presence of mold or bacteria, it cannot resolve distinct cells.
To resolve most kinds of microbial cells, our eyes need assistance; that is, magnification. Magnification reveals the shapes of individual bacteria such as the grape-fermenting bacterium Oenococcus oeni (Fig. 2.3B). Magnifying an object means increasing the object’s apparent dimensions. As the distance increases between points of detail, our eyes can now resolve the object’s shape as a magnified image.
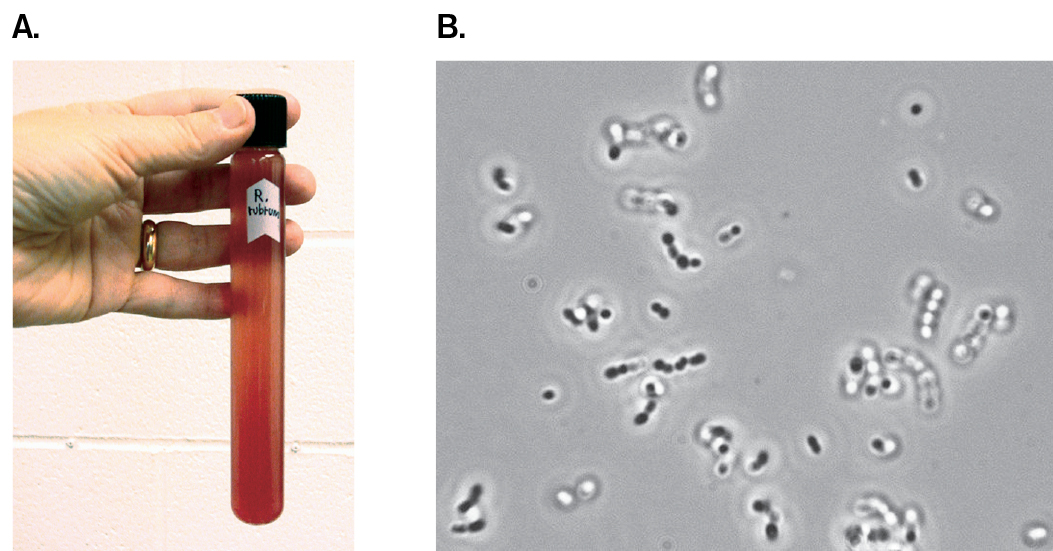
More information
A photo of a bacterial culture tube and a micrograph of coccoid bacteria are shown.
A photo of a human hand holding a tube containing a culture of Rhodospirillum rubrum. The tube contents are turbid and red.
A micrograph of coccoid cells of Oenococcus oeni.
Microbial Size and Shape
Different kinds of microbes differ in size over a range of several orders of magnitude, or powers of ten (Fig. 2.4). Eukaryotic microbes are found across the full range of cell size, from photosynthetic picoeukaryotes abundant in the oceans (0.2–2.0 µm) to giant amebas that reach nearly a centimeter and marine xenophyophores that may reach 20 cm (discussed in Chapter 20).
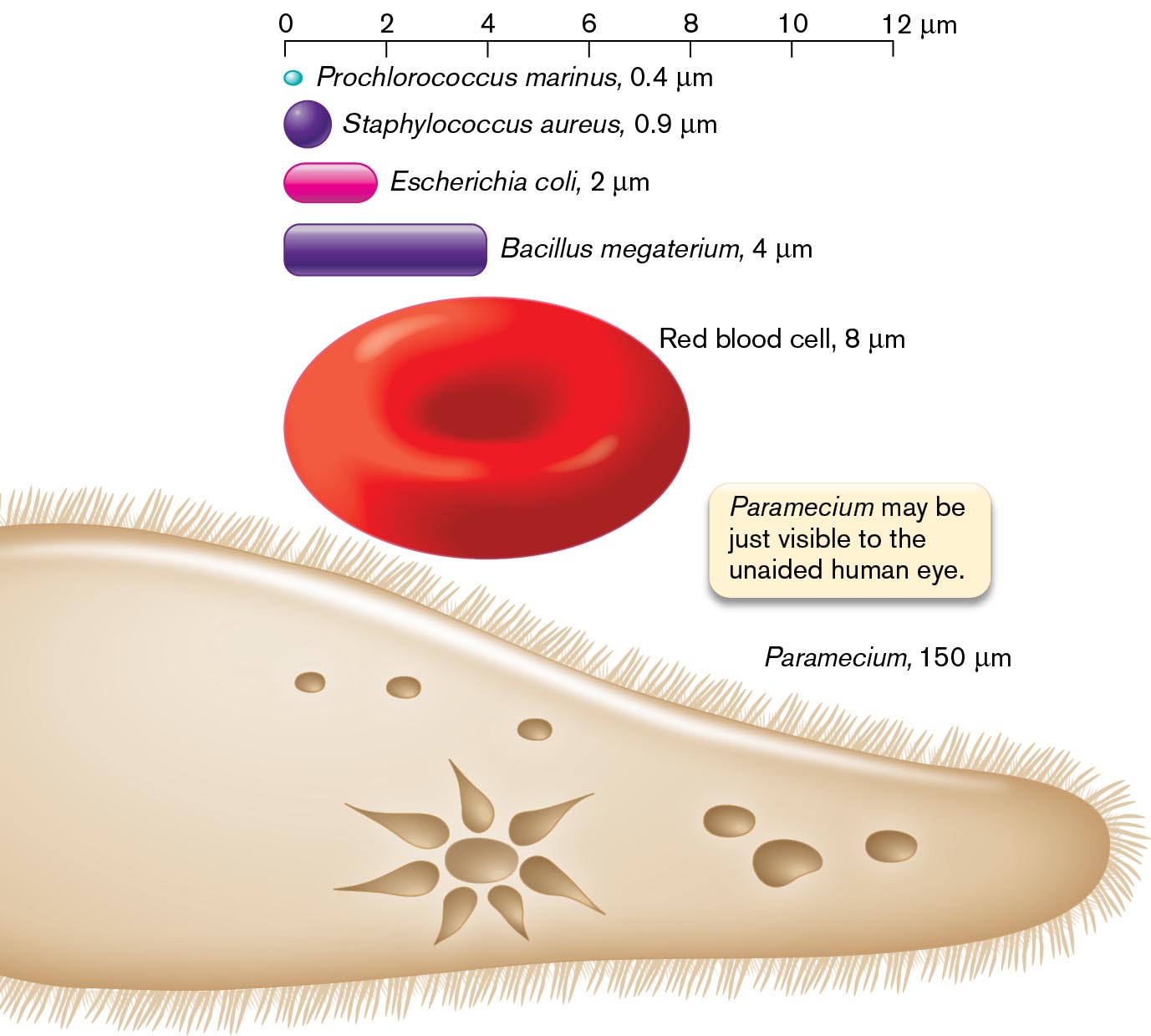
More information
An illustration shows different cell types with different relative sizes. The different cells are as follows: A dot-shaped image labeled as Prochlorococcus Marinus, 0.4 micrometers, a sphere labeled as Staphylococcus aureus, 0.9 micrometers, an oval-shaped cell is labeled as Escherichia coli, 2 micrometers, a rod-shaped cell is labeled as Bacillus megaterium, 4 micrometers, a biconcave disk is labeled as a red blood cell, 8 micrometers, and the ciliated structure of Paramecium at the bottom is 150 micrometers. The corresponding text reads Paramecium may be just visible to the unaided human eye. A scale at the top ranges from 0 to 12 micrometers.
Within a eukaryotic cell, a student’s light microscope may resolve intracellular compartments such as the nucleus and vacuoles containing digested food (Fig. 2.5). Protists show complex shapes and appendages. For example, an ameba from a freshwater ecosystem shows a large nucleus and pseudopods to engulf prey (Fig. 2.5A). Pseudopods can be seen moving by the streaming of their cytoplasm. Another protist readily observed by light microscopy is Trypanosoma brucei, an insect-borne blood parasite that causes African sleeping sickness (Fig. 2.5B). In the trypanosome, we observe a nucleus and a flagellum. Eukaryotic flagella propel the cell by a whiplike action. For more on microbial eukaryotes, see Chapter 20.
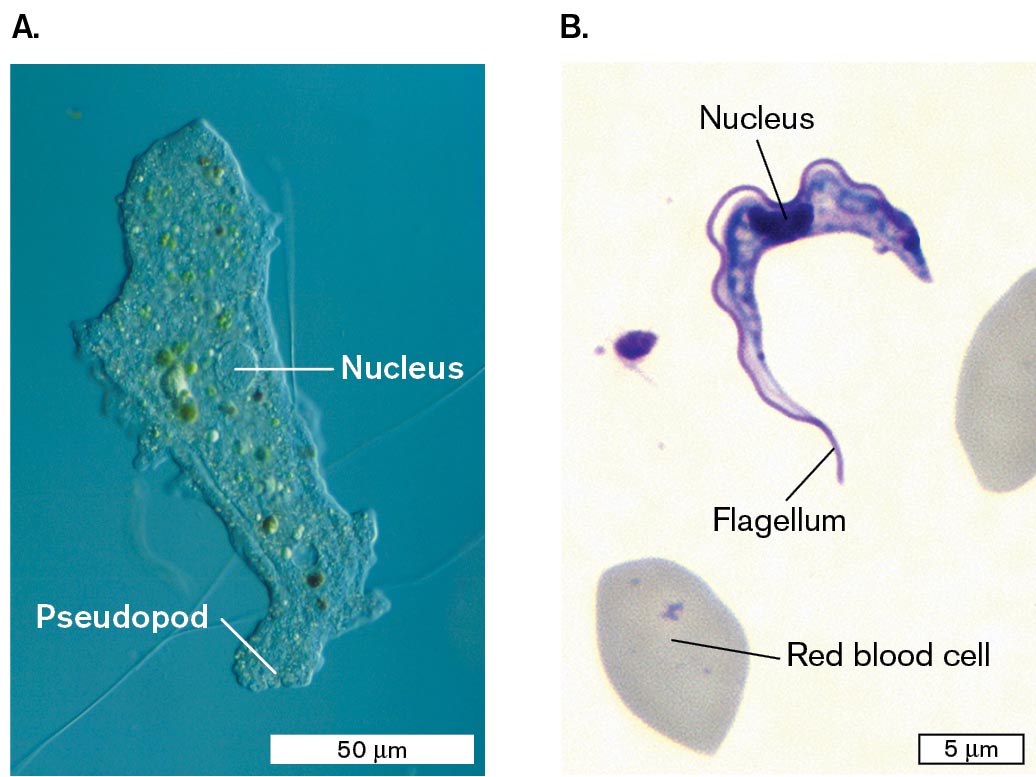
More information
Micrographs of Amoeba proteus and Trypanosoma brucei are shown.
A micrograph of Amoeba proteus under a light microscope. Amoeba proteus has an irregular-shaped structure, and the labeled parts are the nucleus in the center and the extended pseudopod at the bottom. The microbe is 150 micrometers long and about 25 micrometers wide.
A micrograph of Trypanosoma brucei under a light microscope. Trypanosoma brucei consists of an irregularly shaped half-moon structure with a nucleus in the center, and a tail at the bottom labeled as a flagellum. T. brucei is about 20 micrometers long and 2 micrometers wide at its widest point. A red blood cell is identified on the slide near the microbe and appears as a gray oval.
Many prokaryotes (bacteria and archaea) are smaller than 10 µm. Their overall shape can be seen, but most of their internal structures (discussed in Chapter 3) are too small to resolve by light microscopy. Figure 2.6 shows some common cell shapes of bacteria, as visualized by light microscopy or by scanning electron microscopy. With bright-field light microscopy (LM), the cell shape is just discernible under the highest power, usually 1,000× (Fig. 2.6A, C, E). With scanning electron microscopy (SEM), cell shapes appear in greater detail; that is, higher resolution (Fig. 2.6B, D, F). These SEM images are colorized to enhance clarity.
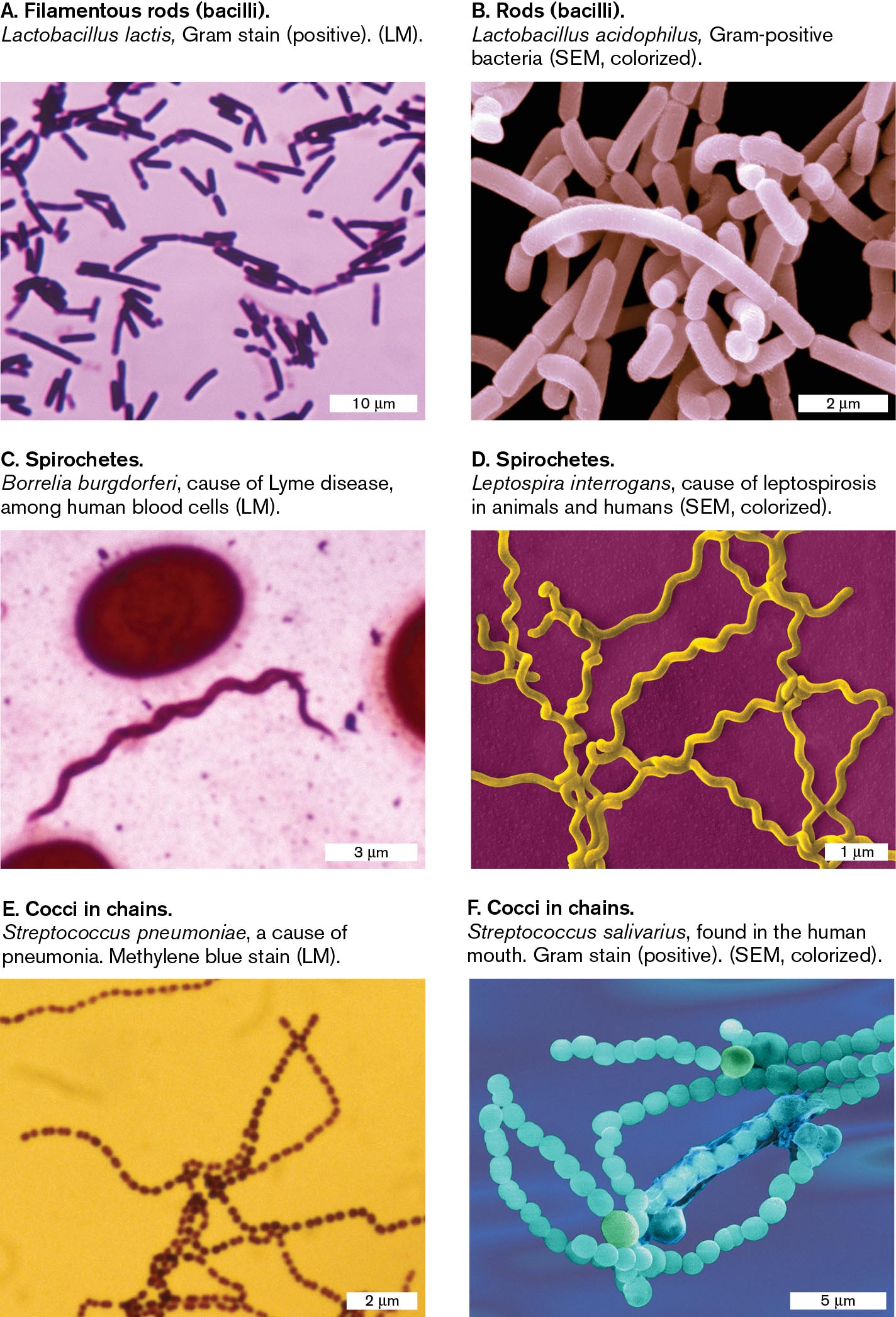
More information
Six micrographs show examples of the common shapes seen in bacterial cells. The first micrograph shows filamentous rods. The second micrograph shows rods. The third and fourth micrographs shows spirochetes. The fifth and sixth micrographs show cocci in chains.
A micrograph of Lactobacillus lactis, a filamentous rod shaped bacteria, as seen under a light microscope. There are many rod-shaped bacteria, each 3-5 micrometers long and 1 micrometer wide. The cells are stained purple.
A micrograph of Lactobacillus acidophilus, a rod shaped bacterium, as seen with a scanning electron microscope. A group of rod shaped bacteria are clumped together. The bacterial cells are each about 2 micrometers long and 0.5 micrometer wide.
A micrograph of Borrelia burgdorferi, a spirochete shaped bacterium, as seen under a light microscope. A single spiral shaped bacterium is on the slide. The cell is about 15 micrometers long and 0.25 micrometer wide. The bacterium is stained pink.
A micrograph of Leptospira interrogans, a spirochete shaped bacterium, as seen with a scanning electron microscope. Several spiral shaped bacteria are seen overlapping each other. The bacteria are about 10 micrometers long and 0.25 micrometer wide.
A micrograph of Streptococcus pneumoniae, a coccus shaped bacterium, as seen under a light microscope. Several long chains of spherical bacteria are seen. Each bacterium is about 0.1 micrometer in diameter.
A micrograph of Streptococcus salivarius, a coccus shaped bacterium, as seen with a scanning electron microscope. Several long chains of spherical bacteria overlap each other. Each bacterium is about 1 micrometer in diameter.
Certain shapes of bacteria are common to many taxonomic groups. For example, both bacteria and archaea form similarly shaped rods, or bacilli (singular, bacillus; Fig. 2.6A and B), and cocci (spheres; singular, coccus; Fig. 2.6E and F). Thus, rods and spherical shapes evolved independently within different taxa. In contrast, an example of a unique bacterial shape that evolved in only one taxon is the spirochete, a tightly coiled spiral (Fig. 2.6C and D). Species of spirochetes cause diseases such as syphilis and Lyme borreliosis. The spiral form of the spirochete cell is maintained by internal axial filaments and flagella, as well as an outer sheath. (For more on spirochetes, see Section 18.5.) A different, unrelated spiral form is the spirillum (plural, spirilla), seen in Figure 2.1. The spirillum is a wide, rigid spiral cell that is similar to a rod-shaped bacillus.
Note: The genus name Bacillus refers to a specific taxonomic group of bacteria, but the term “bacillus” (plural, bacilli) refers to any rod-shaped bacterium or archaeon.
Microscopy at Different Size Scales
To resolve microbes and microbial structures of different sizes requires different kinds of microscopes. Figure 2.7 shows different techniques used to resolve microbes and structures of various sizes. For example, a paramecium can be resolved under a light microscope, but an individual ribosome (20 nm in diameter) requires electron microscopy.
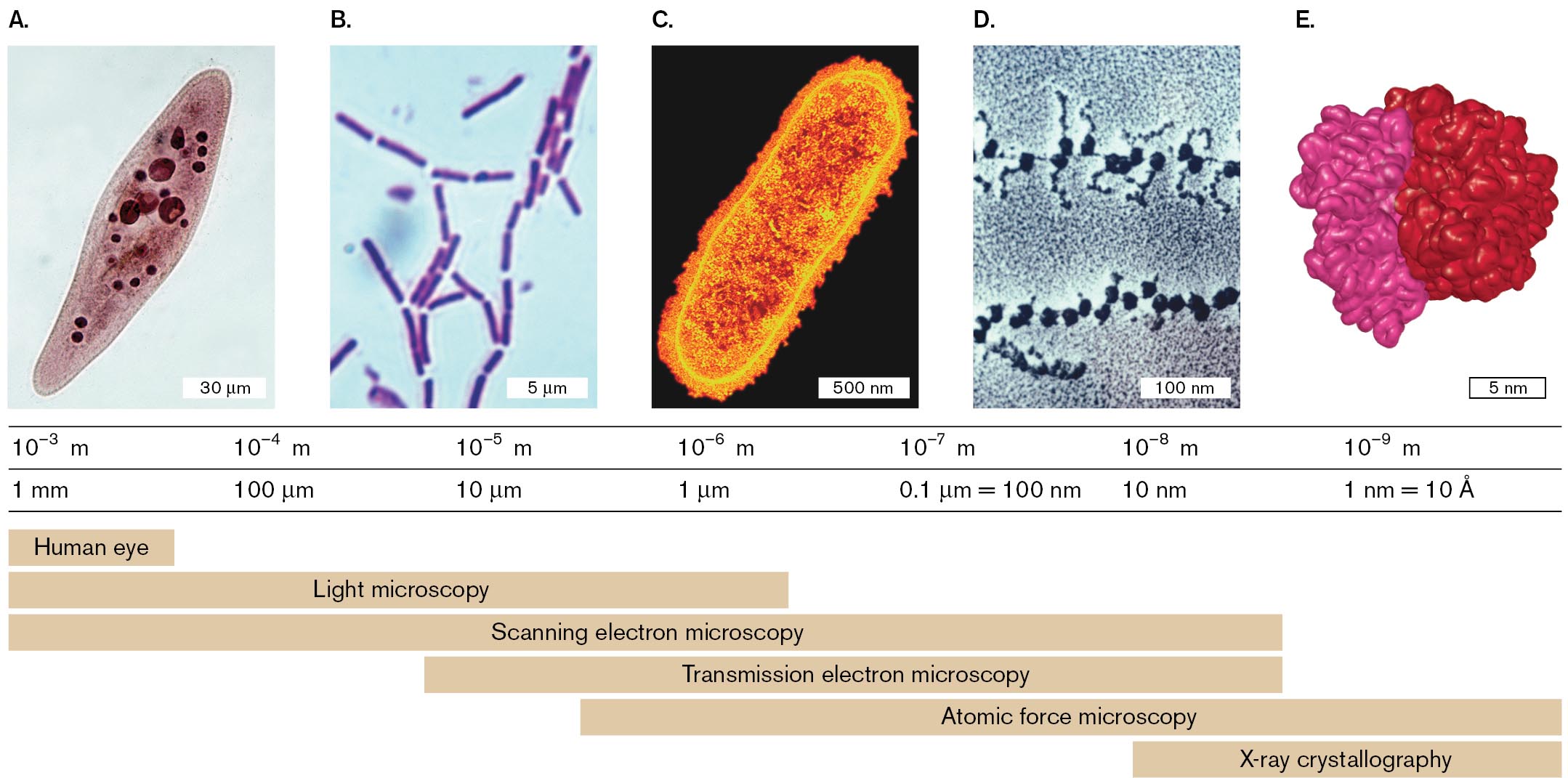
More information
Four micrographs and a model of a ribosome are shown, with corresponding visual ranges and optimal microscope selections. Range of resolution spans from 10 to the negative 3 meter, or 1 millimeter, to 10 to the negative 9 meter, or 1 nanometer. The micrographs and the model serve as examples of subjects that may be visualized within given resolution ranges. The human eye has a range of resolution from 1 millimeter to greater than 100 micrometers. Light microscopy has a range of resolution from 1 millimeter to 1 micrometer. Scanning electron microscopy has a range of resolution from 1 millimeter to 10 nanometers. Transmission electron microscopy has a range of resolution from 10 micrometers to 10 nanometers. Atomic force microscopy has a range of resolution from just under 10 micrometers to 1 nanometer. X-ray crystallography has a range of resolution from 10 nanometers to 1 nanometer.
A micrograph shows a Paramecium, 120 micrometers in length, under a light microscope. The Paramecium is stained so that internal structures are identifiable. This micrograph serves as an example for subjects within the range of resolution from 10 to the negative 3 meter, or 1 millimeter, to 10 to the negative 4 meter, or 100 micrometers. Subjects within this range can be visualized by the human eye, with light microscopy, or with a scanning electron microscope.
A micrograph shows several Bacillus sp. bacteria, each about 5 micrometers in length, under a light microscope. The bacteria are rod-shaped and are stained purple. This micrograph serves as an example for subjects within the range of resolution from 10 to the negative 5 meter, or 10 micrometers, to 10 to the negative 6 meter, or 1 micrometer. Subjects within this range can be visualized with light microscopy, with scanning electron microscopy, or with transmission electron microscopy.
A micrograph shows Escherichia coli, about 1000 nanometers in length, as seen with transmission electron microscopy. E. coli appears as a brightly lit rod shaped bacterium. This micrograph serves as an example for subjects within the range of resolution from 10 to the negative 6 meter, or 1 micrometer, to 10 to the negative 7 meter, or 0.1 micrometer. Subjects within this range can be visualized with light microscopy, scanning electron microscopy, transmission electron microscopy, or atomic force microscopy.
A micrograph shows ribosomes on messenger RNA, each less than 100 nm long, as seen with T E M. The ribosomes are dark circular structures. This micrograph serves as an example for subjects within the range of resolution from 10 to the negative 7 meter, or 0.1 micrometer, to 10 to the negative 8 meter, or 10 nanometers. Subjects within this range can be visualized with scanning electron microscopy, transmission electron microscopy, or atomic force microscopy. Subjects at the smaller end of the range can also be visualized with X-ray crystallography.
A model shows a ribosome, about 10 nanometers in diameter, as seen with X-ray crystallography. Two subunits of the ribosome are identified with color differences. The model serves as an example for subjects within the range of resolution from 10 to the negative 8 meter, or 10 nanometers, to 10 to the negative 9 meter, or 1 nanometer. Subjects within this range can be visualized primarily with X-ray crystallography.
- Light microscopy (LM) resolves images of individual bacteria by their absorption of light. The specimen is commonly viewed as a dark object against a light-filled field, or background; this is called bright-field microscopy (seen in Fig. 2.7A and B). Advanced techniques, based on special properties of light, include phase-contrast and fluorescence microscopy.
- Electron microscopy (EM) uses beams of electrons to resolve details several orders of magnitude smaller than those seen under light microscopy. In scanning electron microscopy (SEM), the electron beam is scattered from the metal-coated surface of an object, generating an appearance of 3D depth. In transmission electron microscopy (TEM; Fig. 2.7C and D), the electron beam travels through the object, where the electrons are absorbed by an electron-dense metal stain.
- Chemical imaging microscopy uses spectrometry to map the chemical contents of a specimen, such as the distribution of nitrogen and carbon compounds.
- X-ray crystallography (also called X-ray diffraction analysis) detects the interference pattern of X-rays entering the crystal lattice of a molecule. From the interference pattern, researchers build a computational model of the structure of the individual molecule, such as a protein or a nucleic acid or even a molecular complex such as a ribosome (Fig. 2.7E).
Thought Question
2.2 (refer to Fig. 2.7) You have discovered a new kind of microbe, never observed before. What kinds of questions about this microbe might be answered by light microscopy? What questions would be better addressed by electron microscopy?
ANSWER
ANSWER
Light microscopy could answer questions such as: What is the overall shape of this cell? Does it form individual cells or chains? Is the organism motile? Only light microscopy can visualize an organism alive. Electron microscopy can answer questions about internal and external subcellular structures. For example, does a bacterial cell possess external filamentous structures, such as flagella or pili? If the dimensions of the unknown microbe are smaller than the lower limits of a light microscope’s resolution, EM may be the only way to observe the organism. Viruses are often characterized by shape, and this shape is observed by electron microscopy.
To Summarize
- Detection is the ability to determine the presence of an object.
- Resolution is the smallest distance by which two objects can be separated and still be distinguished as separate.
- Magnification is an increase in the apparent size of an image.
- Some eukaryotic microbes may be large enough to resolve subcellular structures under a light microscope. Other eukaryotic cells are as small as bacteria.
- Bacteria and archaea are generally too small for subcellular resolution by a light microscope. Their shapes include characteristic forms such as rods and cocci.
- Different kinds of microscopy resolve cells and subcellular structures of different sizes. Chemical imaging microscopy reveals the chemical composition of a cell.
Glossary
- microscope
- A tool that increases the magnification of specimens to enable viewing at higher resolution.
- resolution
- The smallest distance that two objects can be separated and still be distinguished as separate objects.
- focus pl. foci
- The point at which rays of energy converge; in light microscopy, the convergence of light rays maximizes the clarity of the optical image.
- detection
- The ability to determine the presence of an object.
- magnification
- An increase in the apparent size of a viewed object as an optical image.
- bacillus pl. bacilli
- A rod-shaped bacterial or archaeal cell.
- bacillus pl. bacilli
- A rod-shaped bacterial or archaeal cell.
- coccus pl. cocci
- A spherically shaped bacterial or archaeal cell.
- coccus pl. cocci
- A spherically shaped bacterial or archaeal cell.
- spirochete
- A bacterium with a tight, flexible spiral shape; a species of the phylum Spirochetes (Spirochaetota).
- spirillum pl. spirilla
- A rigid, corkscrew-shaped bacterial cell such as Rhodospirillum sp.
- spirillum pl. spirilla
- A rigid, corkscrew-shaped bacterial cell such as Rhodospirillum sp.
- light microscopy (LM)
- Observation of a microscopic object on the basis of light absorption and transmission.
- bright-field microscopy
- A type of light microscopy in which the specimen absorbs light and appears dark against a light background.
- electron microscopy (EM)
- A form of microscopy in which a beam of electrons accelerated through a voltage potential is focused by magnetic lenses onto a specimen.
- scanning electron microscopy (SEM)
- Electron microscopy in which the electron beams scan across the specimen’s surface to reveal the 3D topology of the specimen.
- transmission electron microscopy (TEM)
- Electron microscopy in which electron beams are transmitted through a thin specimen to reveal internal structure.
- TEM
- See transmission electron microscopy.
- chemical imaging microscopy
- A method of microscopy that maps the distribution of specific elements or chemicals within a sample.
- X-ray crystallography or X-ray diffraction analysis
- A technique to determine the positions of atoms (atomic coordinates) within an array of identical molecules or molecular complexes on the basis of the diffraction of X-rays by the molecule.
- X-ray crystallography or X-ray diffraction analysis
- A technique to determine the positions of atoms (atomic coordinates) within an array of identical molecules or molecular complexes on the basis of the diffraction of X-rays by the molecule.
- Figure 2.2:
-
More information
An illustration depicts the principles of human vision. A clear outer layer, the cornea, surrounds the eye. The iris is the colored part of the eye and is slightly more internal than the cornea. The area between cornea and iris is the pupil. More internally is the lens. Along the back lining of the eye is the retina. A tail-like structure at the back of the eye is labeled as the optic nerve, and two fin shaped structures at the sides of the eye are labeled as eye muscle.
A man is standing in front of the eye. A smaller image of the man is mirrored and upside down on the retina. An arrow points toward an illustration describing the retina in detail. The illustration is labeled photoreceptors. Several cones and rod-shaped images are connected between the fovea, a section of closely spaced cone cells.
FIGURE 2.2 ■ Defining the microscopic. Within the human eye, the lens focuses an image on the retina. - Figure 2.1:
-
More information
Two micrographs of methane-oxidizing bacteria are shown, the first at low magnification and the second at high magnification.
The first micrograph shows several spiral shaped bacteria at low magnification. The bacteria are about 4 micrometers long and 0.25 micrometer wide.
The second micrograph shows a bacterium at high magnification with a methane oxidizing structure highlighted. The bacterium is 0.6 micrometer long and 0.1 micrometer wide. Internal structures are visible. A coiled structure is highlighted and described as the intracytoplasmic membranes for methane oxidase.
FIGURE 2.1 ■ Methane-oxidizing bacteria shown by microscopy. A. Phase-contrast microscopy shows the spiral shape of each cell (spirillum). B. Transmission electron microscopy (TEM) reveals intracytoplasmic membranes where methane is oxidized. False color indicates intracellular membranes for methane oxidase.O. V. DANILOVA ET AL. 2016. ISME J. 10:2734–2743O. V. DANILOVA ET AL. 2016. ISME J. 10:2734–2743 - Fig. 2.7:
-
More information
Four micrographs and a model of a ribosome are shown, with corresponding visual ranges and optimal microscope selections. Range of resolution spans from 10 to the negative 3 meter, or 1 millimeter, to 10 to the negative 9 meter, or 1 nanometer. The micrographs and the model serve as examples of subjects that may be visualized within given resolution ranges. The human eye has a range of resolution from 1 millimeter to greater than 100 micrometers. Light microscopy has a range of resolution from 1 millimeter to 1 micrometer. Scanning electron microscopy has a range of resolution from 1 millimeter to 10 nanometers. Transmission electron microscopy has a range of resolution from 10 micrometers to 10 nanometers. Atomic force microscopy has a range of resolution from just under 10 micrometers to 1 nanometer. X-ray crystallography has a range of resolution from 10 nanometers to 1 nanometer.
A micrograph shows a Paramecium, 120 micrometers in length, under a light microscope. The Paramecium is stained so that internal structures are identifiable. This micrograph serves as an example for subjects within the range of resolution from 10 to the negative 3 meter, or 1 millimeter, to 10 to the negative 4 meter, or 100 micrometers. Subjects within this range can be visualized by the human eye, with light microscopy, or with a scanning electron microscope.
A micrograph shows several Bacillus sp. bacteria, each about 5 micrometers in length, under a light microscope. The bacteria are rod-shaped and are stained purple. This micrograph serves as an example for subjects within the range of resolution from 10 to the negative 5 meter, or 10 micrometers, to 10 to the negative 6 meter, or 1 micrometer. Subjects within this range can be visualized with light microscopy, with scanning electron microscopy, or with transmission electron microscopy.
A micrograph shows Escherichia coli, about 1000 nanometers in length, as seen with transmission electron microscopy. E. coli appears as a brightly lit rod shaped bacterium. This micrograph serves as an example for subjects within the range of resolution from 10 to the negative 6 meter, or 1 micrometer, to 10 to the negative 7 meter, or 0.1 micrometer. Subjects within this range can be visualized with light microscopy, scanning electron microscopy, transmission electron microscopy, or atomic force microscopy.
A micrograph shows ribosomes on messenger RNA, each less than 100 nm long, as seen with T E M. The ribosomes are dark circular structures. This micrograph serves as an example for subjects within the range of resolution from 10 to the negative 7 meter, or 0.1 micrometer, to 10 to the negative 8 meter, or 10 nanometers. Subjects within this range can be visualized with scanning electron microscopy, transmission electron microscopy, or atomic force microscopy. Subjects at the smaller end of the range can also be visualized with X-ray crystallography.
A model shows a ribosome, about 10 nanometers in diameter, as seen with X-ray crystallography. Two subunits of the ribosome are identified with color differences. The model serves as an example for subjects within the range of resolution from 10 to the negative 8 meter, or 10 nanometers, to 10 to the negative 9 meter, or 1 nanometer. Subjects within this range can be visualized primarily with X-ray crystallography.
FIGURE 2.7 ■ Microscopy and X-ray crystallography, range of resolution. A. Paramecium (stained light microscopy, LM). B. Bacillus sp. (stained LM). C. Escherichia coli (transmission electron microscopy, TEM). D. Ribosomes on messenger RNA (TEM). E. Ribosome model (X-ray crystallography).ED RESCHKE/PETER ARNOLD, INC./GETTY IMAGESBIODISC/VISUALS UNLIMITEDT. J. BEVERIDGE/VISUALS UNLIMITEDKISELEVA & DONALD FAWCETT/VISUALS UNLIMITED